13 Neurotransmitter Identification and Action: Ionotropic Receptors versus Metabotropic Receptors
Neurotransmitters are chemicals that are released at the synapse. In this chapter, we will introduce basic information about neurotransmitters and how they are identified. Next, an overview of neurotransmitter receptors will be provided.
Receptors are proteins located on the postsynaptic cell that are capable of sending a signal to change the function or activity of the postsynaptic neuron. Most receptors that function in neurotransmission are large transmembrane proteins. On the extracellular surface of the protein is a specific series of amino acid residues called the active site. The active site, also called the orthosteric site, is shaped to allow molecules of neurotransmitter to bind to the receptor. Receptors are classified into one of two main categories: Ionotropic receptors and Metabotropic receptors (G-protein coupled receptors).
Neurotransmitters
Neurotransmitters are the chemicals released at the presynaptic terminal that then bind to the postsynaptic cell. A neurotransmitter system is the neurotransmitter and everything needed for the synthesis of the transmitter, the packaging of the transmitter into vesicles, the proteins necessary for reuptake of the transmitter into the presynaptic cell, degradation of the transmitter, and postsynaptic signaling of the transmitter. In the following chapters, we will discuss various neurotransmitter systems.
Though there are neurons that can release more than one type of neurotransmitter (referred to as co-transmitters), most neurons release just one type of neurotransmitter, allowing neurons to be identified by the neurotransmitter they release (e.g. dopaminergic neurons). The different neurotransmitters can be separated into three different chemical categories:
- Amino Acids (Glutamate, GABA, Glycine)
- Amines (Acetylcholine, Dopamine, Epinephrine, Histamine, Norepinephrine, Serotonin)
- Peptides (Dynorphin, Enkephalin, Substance P, Neuropeptide Y)
To identify whether a chemical can be classified as a neurotransmitter, it must meet the following requirements:
- The chemical has to be stored or located within the presynaptic neuron. Both immunohistochemistry and immunocytochemistry can use antibodies directed against a specific protein important for neurotransmitter synthesis or storage to localize the protein to a neuron and determine whether a neuron has the appropriate machinery to make the neurotransmitter and thus likely release the neurotransmitter.
- The chemical has to be released from the presynaptic neuron following appropriate stimulation.
- When the chemical is applied to the postsynaptic cell by an experimenter, the response of the postsynaptic cell should be similar to the response following normal release from the presynaptic cell.
Neurotransmitters can be classified on their function as either ‘excitatory’ or ‘inhibitory’. In the following chapters, we will see that glutamate is an example of an excitatory neurotransmitter. This is because the binding of glutamate to the postsynaptic cell typically generates excitatory postsynaptic potentials, which makes the inside of the cell closer to zero and thus closer to threshold potential for the cell, increasing the likelihood of firing an action potential. GABA and Glycine, however, are typically considered as inhibitory neurotransmitters. This is because the binding of either GABA or glycine to the postsynaptic cell typically generates inhibitory postsynaptic potentials, which makes the inside of the cell more negative and further from threshold potential of the cell, decreasing the likelihood of firing an action potential.
Most neurotransmitters, however, can produced either excitatory postsynaptic potentials or inhibitory postsynaptic potentials depending on the properties of the postsynaptic receptor that they bind to. Neurotransmitters each bind to specific receptors that produce specific postsynaptic responses.
The subsequent chapters will review the main neurotransmitters including their synthesis, storage, and their receptors.
Neurotransmitter Receptors
Ionotropic Receptors
Ionotropic receptors are also called neurotransmitter-gated or ligand-gated channels. They are ion channels that open in response to the binding of a neurotransmitter. They are primarily located along the dendrites or cell body, but they can be present anywhere along the neuron if there is a synapse. Ionotropic receptors are important for receiving incoming information from other neurons.
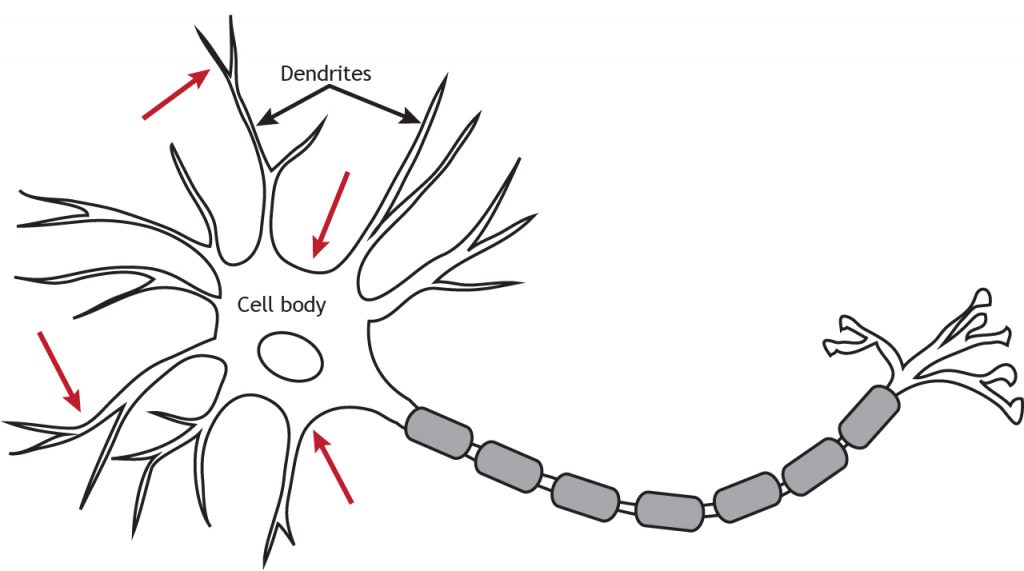
Physically, ionotropic receptors are transmembrane proteins with a large-diameter pore through which ions can pass. Although ionotropic receptors are ion channels, they open in a different way than the voltage-gated ion channels needed for propagation of the action potential. The ionotropic receptors are ligand-gated, which means that a specific molecule, such as a neurotransmitter, must bind to the receptor to cause the channel to open and allow ion flow. As seen in previous chapters, the voltage-gated channels open in response to the membrane potential reaching threshold (a specific voltage).
Ionotopic channels are often said to use a direct signaling mechanism because the neurotransmitter binds directly to the ion channel that it is going to open.
Animation 13.1. Ionotropic receptors, also called ligand-gated channels, are ion channels that are opened by the binding of neurotransmitters. Voltage-gated channels are opened by the membrane potential of the cell reaching threshold. Both types of channels allow ions to diffuse down their electrochemical gradient. The lined, teal channels represent glutamate receptors; the solid yellow channels represent GABA receptors; the dotted, blue channels represent voltage-gated sodium channels. ‘Ion Channel Gating’ by Casey Henley is licensed under a Creative Commons Attribution Non-Commercial Share-Alike (CC-BY-NC-SA) 4.0 International License. View static image of animation.
These channels only open when a specific ligand binds to the active site on the extracellular side of the protein. Neurotransmitters and receptors fit together like a lock and key; only certain neurotransmitters are able to bind to and open certain receptors.
Once a neurotransmitter activates the ionotropic receptor, ions will move through the channel based on the electrochemical gradient for that ion. As a result of ion movement, the cell’s membrane potential will change. For example, if the ionotropic channel allowed for movement of sodium, the electrochemical gradient for sodium will promote sodium rushing into the cell, causing the inside of the cell to get more positive (decrease in membrane potential).
Ionotropic receptors are able to induce a change in membrane potential very rapidly, on the scale of milliseconds. Due to the nature of the amino acid residues that make up the pore of ionotropic receptors, they can be very selective for certain ions. For example, negatively charged residues lining the inside of the pore repel negatively charged Cl– ions while allowing positively charged cations to pass through the channel.
Animation 13.2. Since neurotransmitter receptors can only bind specific neurotransmitters, glutamate binds to and opens glutamate receptors, but has no effect on GABA receptors. The lined, teal channels represent glutamate receptors; the solid yellow channels represent GABA receptors. ‘Ligand and Receptor’ by Casey Henley is licensed under a Creative Commons Attribution Non-Commercial Share-Alike (CC-BY-NC-SA) 4.0 International License. View static image of animation.
Metabotropic Receptors (G-protein-coupled receptors)
Metabotropic receptors (also called G-protein-coupled receptors [GPCRs]), are membrane-bound proteins. These receptor complexes cause the cell to change its metabolism in a way that leads to either excitation or inhibition of the postsynaptic cell. Like ionotropic receptors, metabotropic receptors are primarily located along the dendrites or cell body, but they can be present anywhere along the neuron if there is a synapse.
Unlike ionotropic receptors (direct mechanism), ions do not pass through GPCRs. Instead, metabotropic receptors use the actions of G proteins, proteins which induce changes in neuronal excitability through the action of second messenger signaling molecules. Due to these extra steps, GPCRs have slower effects than ionotropic receptors, but they can have long-lasting effects, unlike the brief action of a postsynaptic potential.
G-Proteins
G-proteins are enzymes with three subunits: alpha, beta, and gamma. There are multiple types of alpha subunits (Gαs, Gαi, Gαq), and each initiate different cellular cascades in the neuron.
Functionally speaking, these G-proteins are capable of binding to molecules of guanosine triphosphate (GTP) or guanosine diphosphate (GDP). Chemically similar to ATP, GTP can function as a source of energy. G-proteins themselves exhibit catalytic activity of GTP. This means that they are capable of breaking down GTP into the less-energetic GDP. When GTP is bound to the GPCR, the receptor is active. When this molecule is hydrolyzed into GDP, the receptor becomes inactive.
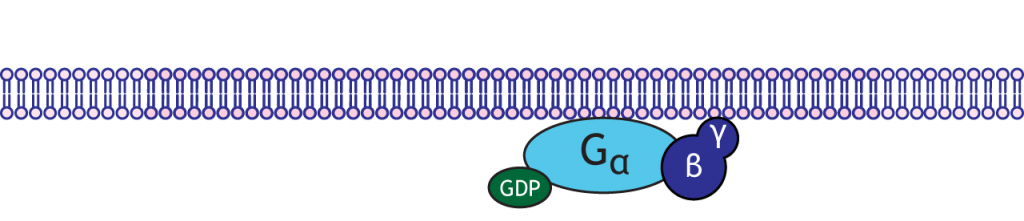
Metabotropic Receptors
A metabotropic receptor or GPCR is a transmembrane protein that has an extracellular binding site for a neurotransmitter. Metabotropic receptors are physically linked to G-proteins, which exist on the inner surface of the cell membrane.
When a neurotransmitter binds to a GPCR it undergoes a conformational change, which allows the receptor to interact with an associated inactivated G-protein complex.
The complex that binds is specific to the receptor; different metabotropic receptors for the same neurotransmitter can have different effects in the cell due to which G-protein binds. Once coupled to the receptor, the GDP molecule is exchanged for a GTP molecule and the G-protein becomes activated.
After activation, the G-protein complex will separate into the alpha-GTP subunit and the beta-gamma subunit. Both components can alter the function of effector proteins in the cell. Effector protein functions can range from altering ion permeability across the membrane by opening ion channels to initiating second messenger cascades. Second messenger cascades can have long-term, widespread, and diverse cellular effects including activation of cellular enzymes or altering gene transcription.
Animation 13.4. Once activated, the G-protein complex will separate into the alpha-GTP subunit and the beta-gamma subunit. These subunits can stimulate or inhibit effector proteins within the cell. ‘G-protein Effects’ by Casey Henley is licensed under a Creative Commons Attribution Non-Commercial Share-Alike (CC BY-NC-SA) 4.0 International License. View static image of anim[latex]null[/latex]ation.
Adenylyl Cyclase / cAMP Second Messenger Cascade
The cyclic AMP (cAMP) second messenger pathway is used by many GPCRs. When a neurotransmitter binds to a GPCR, it causes activation of the Gαs alpha subunit. The Gαs subunit (“s” for stimulatory) translocates within the cell to stimulate an effector enzyme called adenylyl cyclase. When activated, adenylyl cyclase converts ATP to cAMP in the cytoplasm. cAMP is considered a 2nd messenger (the 1st messenger was the neurotransmitter). As a second messenger, cAMP has the ability to alter proteins inside the cell. Specifically, cAMP activates another enzyme called protein kinase A (PKA) by binding to the regulatory subunits, allowing the catalytic (functional) subunits to separate and become active. PKA is a kinase, a group of enzymes that add a phosphate molecule to proteins, a mechanism called phosphorylation. The addition of the phosphate changes the activity of the protein and how it functions in the cell. Typically, phosphorylation activates proteins within the cell.
Animation 13.5. GPCRs that couple to the Gs alpha subunit initiate the adenylyl cyclase / cAMP pathway. The Gs subunit activates adenylyl cyclase, which then converts ATP to cAMP. cAMP binds to and activates protein kinase A (PKA), which phosphorylates proteins in the cell. ‘Adenylyl Cyclase Pathway’ by Casey Henley is licensed under a Creative Commons Attribution Non-Commercial Share-Alike (CC BY-NC-SA) 4.0 International License. View static image of animation.
The end effects of this pathway will depend on which proteins are targeted. For example, cAMP can gate ion channels and PKA can phosphorylate ion channels altering permeability and membrane potential. Phosphorylation can open the channel, or it may modulate the activity of the channel, making the channel easier to open or remain open longer.
Animation 13.6. The adenylyl cyclase / cAMP pathway can alter many cellular functions. One example is that both cAMP and PKA can open ion channels. Like ligand-gated channels, there are also cAMP-gated channels, which open after cAMP binding. PKA is able to phosphorylate and modulate ion channel function by converting ATP to ADP. ‘Second Messenger Ion Channel Action’ by Casey Henley is licensed under a Creative Commons Attribution Non-Commercial Share-Alike (CC BY-NC-SA) 4.0 International License. View static image of animation.
In addition to altering ion channel function, PKA can phosphorylate other proteins important for neuron function, such as proteins involved with neurotransmitter synthesis and release. One other critical target of PKA phosphorylation is the transcription factor CREB (cAMP response element-binding protein). Transcription factors bind to DNA in the nucleus and change the rate of gene transcription. Phosphorylation by PKA can cause CREB to initiate transcription of genes, creating new proteins for the neuron. Depending on which genes are transcribed, the effects on the neuron can be long-lasting.
Overall, neurotransmitters working through GPCRs and second messenger cascades (like the adenylyl cyclase pathway) can cause a diverse range of cellular effects: from opening ion channels, to changing protein activity via phosphorylation, to altering the proteins synthesized in the neuron.
Animation 13.7. PKA can phosphorylate a number of proteins involved with neuron function. It can target proteins involved with neurotransmitter synthesis, packing, and release, or it can enter the nucleus and phosphorylate CREB, a transcription factor that can initiate gene transcription and protein synthesis. ‘PKA Targets’ by Casey Henley is licensed under a Creative Commons Attribution Non-Commercial Share-Alike (CC BY-NC-SA) 4.0 International License. View static image of animation.
Alternatively, a GPCR that is coupled with Gαi causes a decrease in excitability. In many ways, Gαi proteins serve the opposite function as Gαs proteins—the “i” stands for inhibitory. Whereas activation of Gαs increases the action of adenylyl cyclase, Gαi proteins decrease adenylyl cyclase activity. Therefore, Gαi activation decreases the intracellular concentration of cAMP, in turn decreasing PKA activity. Given the function of PKA as a kinase that increases cellular excitation as described above, a GPCR coupled to Gαi that causes decreased PKA activity inhibits cellular activity through multiple mechanisms, some of which include decreased current through receptors, decreased trafficking of receptors to the presynaptic neuronal membrane, and decreased transcription of certain genes.
Phosphlipase C / IP3 / DAG Second Messenger Cascade
Generally, Gαq is an excitatory G-protein alpha subunit. The Gαq subunit initiates a separate signaling pathway in the cell by activating the effector enzyme phospholipase C. Phospholipase C targets PIP2 (phosphatidylinositol 4,5-bisphosphate), which is a phospholipid present in the plasma membrane of the cell. PLC will split PIP2 into two separate second messenger molecules: the soluble IP3 (inositol 1,4,5-trisphosphate) and membrane-embedded DAG (diacylglycerol).
Animation 13.8. The Gq G-protein subunit activates phospholipase C, which converts the phospholipid PIP2 in the cell membrane into DAG, another membrane-bound molecule, and IP3, a cytoplasmic molecule. DAG can interact with PKA, initiating phosphorylation of cellular proteins. IP3 opens calcium channels in the endoplasmic reticulum, allowing calcium to flow into the cytoplasm. Calcium, another second messenger can have many cellular effects. It can bind to calmodulin, which then activates CaMK, causing phosphorylation of more protein targets. ‘IP3-DAG Pathway’ by Casey Henley is licensed under a Creative Commons Attribution Non-Commercial Share-Alike (CC BY-NC-SA) 4.0 International License. View static image of animation.
DAG remains in the membrane and interacts with protein kinase C (PKC). PKC is an enzyme that can act to increase neurotransmitter release probability.
One function of IP3 is to move to the endoplasmic reticulum, where it opens calcium channels embedded in the endoplasmic reticulum and allows calcium to flow into the cytosol, elevating intracellular calcium levels. This increase in calcium concentration can depolarize the cell and activate calcium-dependent processes, which often lead to cellular excitation.
Calcium also acts as a second messenger in the cell. One important effect is the binding of calcium to the calmodulin protein. This complex can then activate another kinase, the calcium/calmodulin-dependent protein kinase (CaMK). Both PKC and CaMK can phosphorylate specific cellular and nuclear proteins like PKA.
So, depending on the type of Gα subunit that the G-protein is associated with, there will be different outcomes for the cell. In general, Gαs will “stimulate” the cell by activating adenylyl cyclase. Gαi will “inhibit” the cell by inhibiting adenylyl cyclase. Gαq will “stimulate” the cell by activating phospholipase C.
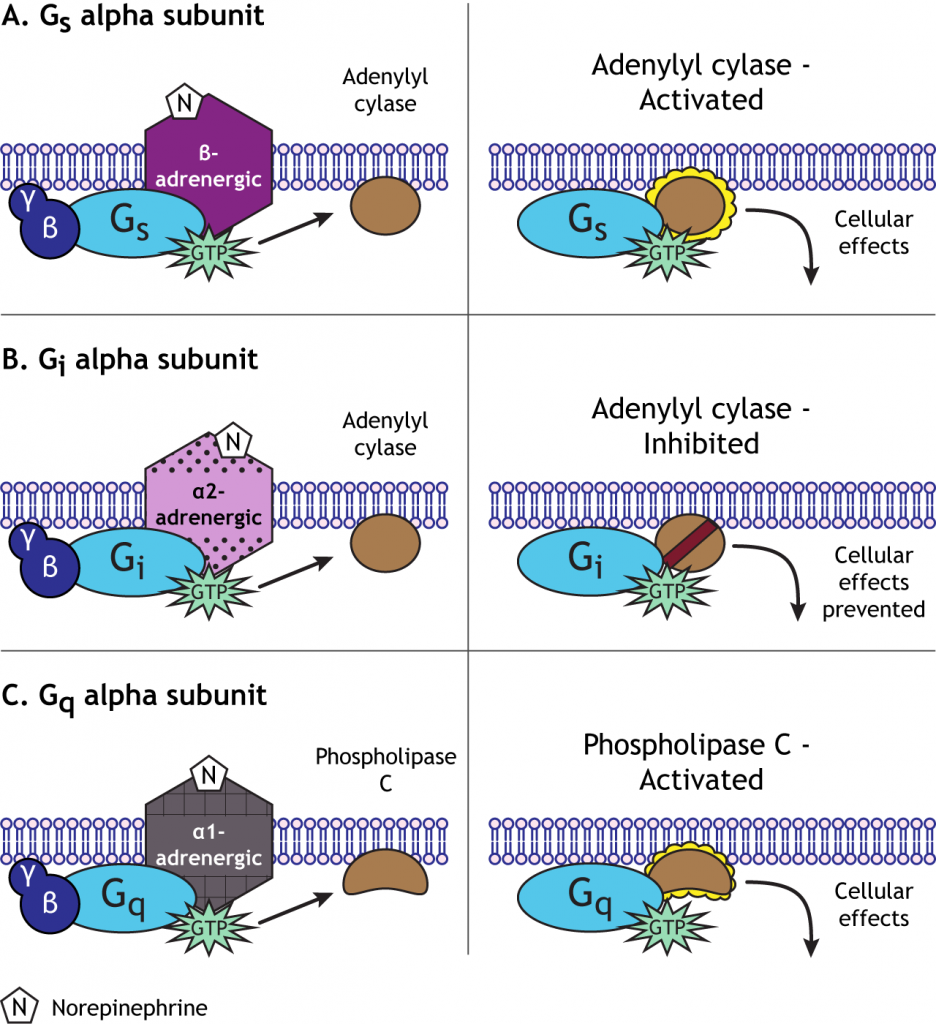
Signal Amplification
One characteristic of GPCR activation is the signal amplification that takes place. One receptor is able to activate more than one G-protein complex. The effector protein activated by the G-protein can create many second messengers, and the activated protein kinases can each phosphorylate multiple cellular proteins. This means that one neurotransmitter can have a significant effect on cellular function.
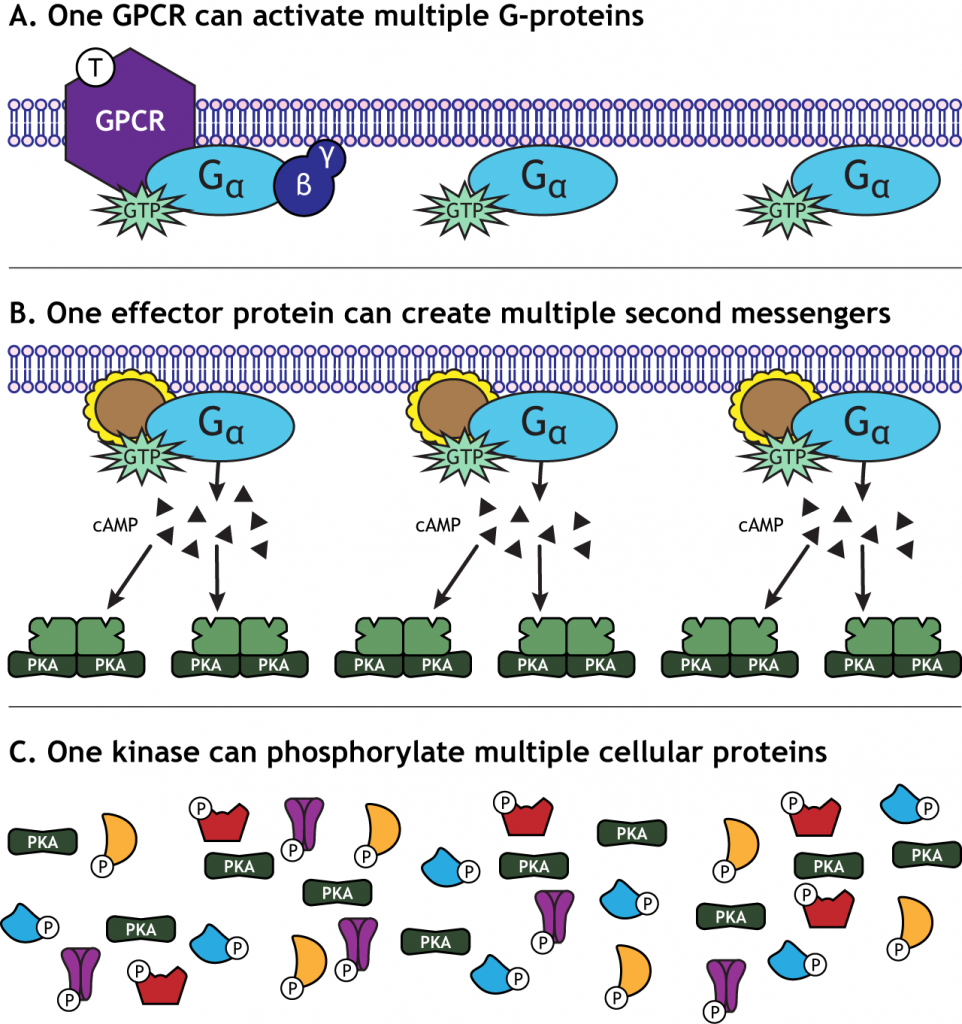
Signal Termination
Eventually, the cascade initiated by binding of the neurotransmitter to the GPCR needs to end. The alpha subunit of the G-protein is able to convert the bound GTP back to GDP after a short period of time, inactivating the G-protein. The alpha subunit will then interact with a beta-gamma subunit and stay in the resting state until activated by another GPCR. Enzymes in the cell called protein phosphatases find and remove the phosphate groups added to cellular proteins by the protein kinases. Finally, other cellular mechanisms exist to remove calcium from the cytoplasm and degrade other second messengers.
Key Takeaways
- Ionotropic receptors are ligand-gated ion channels that open when a specific neurotransmitter binds
- Ionotropic receptors represent a direct mechanism to alter the postsynaptic cell because the neurotransmitter binds to the ion channel directly
- Metabotropic receptors represent an indirect mechanisms to alter the postsynaptic cell because the neurotransmitters binds to a separate protein from the effector proteins that alter the postsynaptic cell
- G-protein-coupled receptors rely on the activation of G-proteins to cause cellular changes
- G-protein-coupled receptors have slower effects than ligand-gated receptors
- G-proteins can open ion channels, alter protein function via phosphorylation, and alter gene transcription
- The Gs subunit initiates the adenylyl cyclase / cAMP signaling pathway
- The Gi subunit inhibits the adenylyl cyclase / cAMP signaling pathway
- The Gq subunit initiates the phospholipase C / IP3 / DAG signaling pathway
Test Yourself!
Attributions
Portions of this chapter were remixed and revised from the following sources:
- Foundations of Neuroscience by Casey Henley. The original work is licensed under a Creative Commons Attribution-NonCommercial-ShareAlike 4.0 International License
- Open Neuroscience Initiative by Austin Lim. The original work is licensed under a Creative Commons Attribution-NonCommercial 4.0 International License.
Postsynaptic receptors in which neurotransmitters bind and cause the opening of an ion channel. The ion channel and receptor are the same transmembrane protein.
Postsynaptic receptors in which neurotransmitters bind and cause the activation of an associated G-protein and cell signaling cascades. The affected ion channel and receptor are found on different transmembrane proteins..
a specific membrane potential value that is more positive than resting membrane potential. Threshold potential can differ between cells.
enzyme that converts ATP into cAMP
an enzyme that phosphorylates targets (adds a phosphate group) that typically activates the target
a protein that controls the rate of transcription by binding to specific DNA sequences
cellular structure that stores as an intracellular calcium storage site
enzyme responsible for removing phosphate groups from targets, typically inactivating targets