32 Auditory System: Central Processing
All sensory systems follow the same general path of communication into our nervous systems and awareness. First, the incoming signal must reach a that can change its electrical properties in response to the stimulus. Then, that information initiates a series of signals into the CNS, reaching structures such as the thalamus, primary sensory cortical areas, and finally, higher order perception. Although there are several sensory components throughout our body that detect these signals, there are no sensory receptors in our central nervous system. We previously saw this pattern for the visual system, and now we’ll see it mirrored in many ways by the auditory system. For the auditory system, following the activation of hair cells within the cochlea, sound information is transmitted to brain structures including the thalamus and cortex for processing to be perceived as sounds.
Central Auditory Pathway
Hair cells within the cochlea form glutamatergic synapses onto spiral ganglion cells. The axons of these neurons make up the vestibulocochlear nerve (Cranial nerve VIII). Neurons project to and synapse on neurons within the ipsilateral cochlear nuclei of the medulla. Because the medulla neurons only process sound from one ear, these cells are referred to as monaural. (one ear).
The cochlear nuclei neurons within the medulla have axons that split and synapse on both the ipsilateral and contralateral superior olive within the pons. The superior olive is the first structure that processes sound information from both ears, so it is referred to as binaural. All remaining structures in the central auditory pathway process sounds from both ears, and thus are also binaural.
From the pons, neurons travel via the lateral lemniscus (a lemniscus is a collection of axons) and then synapse on the inferior colliculus of the midbrain tectum. Signaling within the inferior colliculus is important for interactions between multiple sensory inputs and a motor response. These inferior colliculus neurons are particularly responsive to biologically-relevant sounds, such as unexpected noises, which may signal an approaching predator. Processing in the inferior colliculus helps the animal focus their attention on these stimuli.
The inferior colliculus then conveys that auditory information into the medial geniculate nucleus, one of the nuclei of the thalamus. These thalamic neurons then send projections into the primary auditory cortex located dorsally in the temporal lobe.
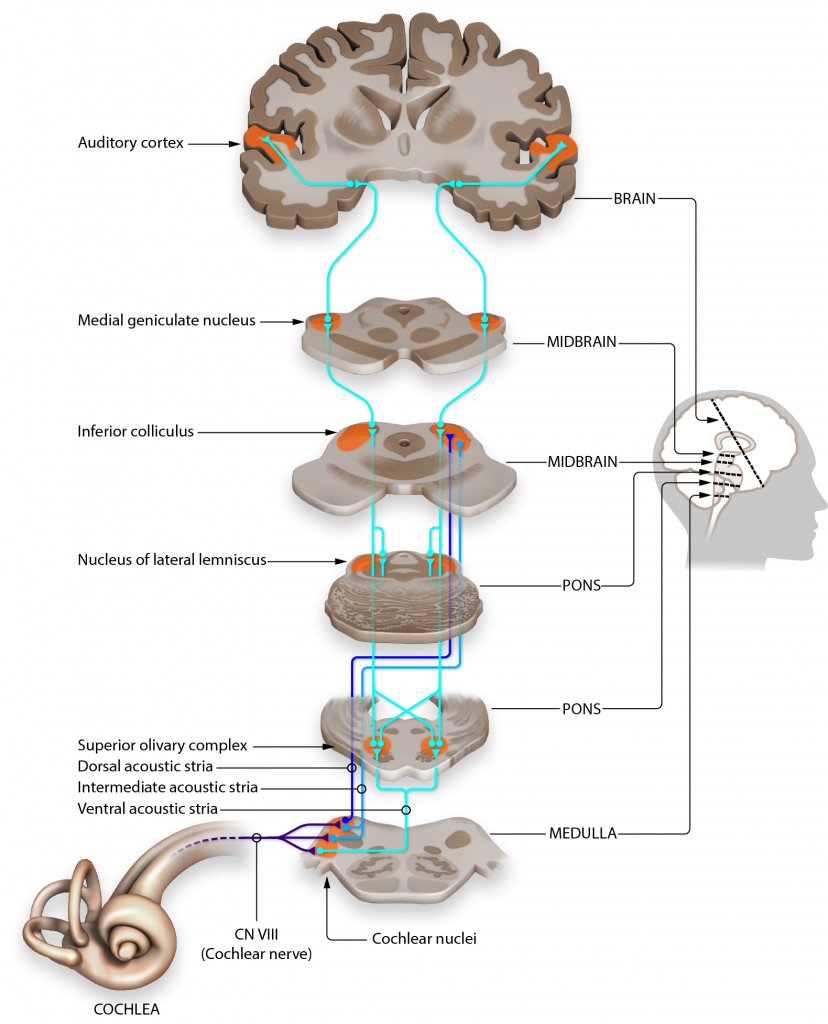
Tonotopy
The structures responsible for the central processing of sound, including the basilar membrane, the spiral ganglion, the cochlear nucleus, the inferior colliculus, and the auditory cortex are tonotopically organized, meaning that adjacent physical areas are responsible for conveying information from adjacent frequencies. For example, the hair cells that respond most to 440 Hz vibrations are right next to cells that respond maximally to 441 Hz, but far away from cells that respond most to 14,000 Hz. Likewise, in the auditory cortex, the cells that best process 440 Hz are adjacent to those that best process 441 Hz, but far away from those that maximally respond to 14,000 Hz.
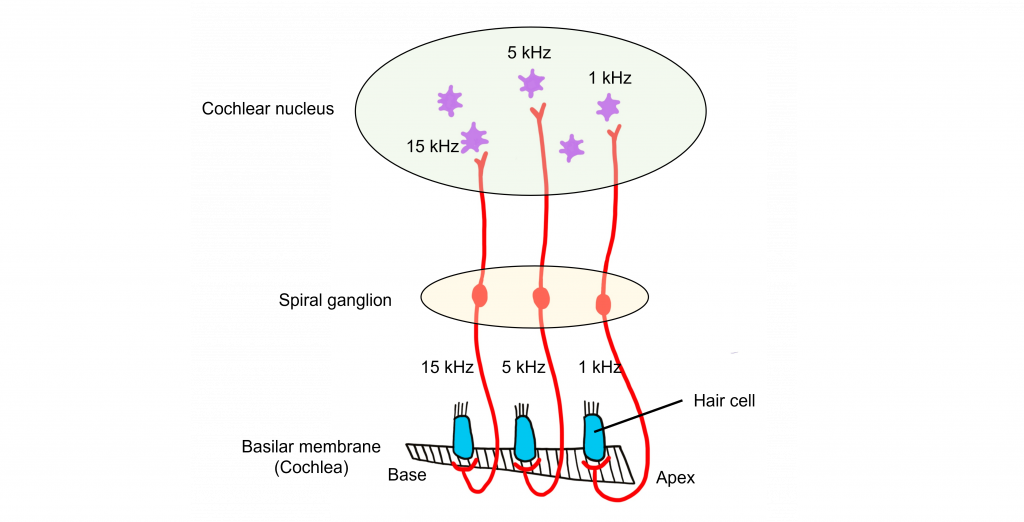
Auditory Cortex
The auditory cortex (also called A1) is located within the temporal lobes in both hemispheres of the brain. Similar to the visual cortex, the auditory cortex is also made up of 6 layers of cells that have columnar organization. Each cortical column responds to a specific frequency. The auditory cortex is tonotopically organized like the other structures in the central auditory pathway. It is organized as isofrequency bands, like strips, that respond to relatively the same frequency.
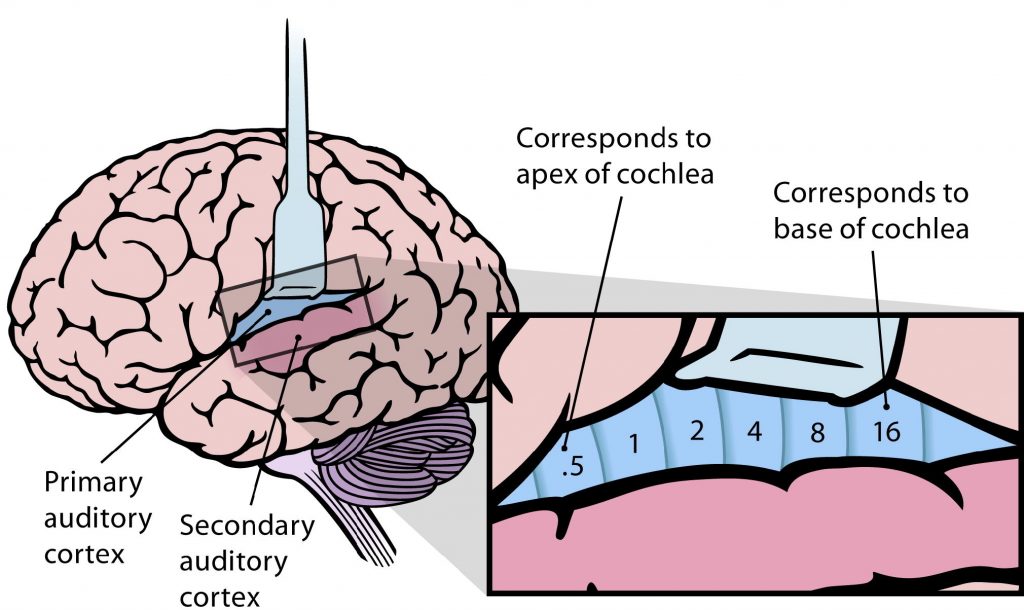
Sound Localization
Sound localization is an important function for animals. We can localize sounds in the horizontal plane and the vertical plane via different mechanisms.
Localizing Sounds in the Horizontal Plane
When considering how we localize sounds in the horizontal plane, we assume that the sound does not change in elevation. As humans, our ears are located on the sides of our heads, which means that sounds will arrive at our ears at different times depending on the origin of the sound. The time between when sound reaches the first ear until the time it reaches the second ear is referred to as the interaural time difference (ITD).
If we assume that there are 20 cm between the two ears of a human, then we can determine the ITD for the left ear. If a sound is coming directly from the right, then there will be a 0.6 msec ITD for the left ear. If sounds are coming from directly in front of a person, then the time that it takes to reach the right ear will be exactly the same as the time that it takes to reach the left ear, thus there is no ITD (0 msec). If we consider an intermediary angle of sound origin coming from 45° we can determine that the ITD will be 0.3 msec, exactly between the values from when sounds originate directly in front and directly to the right.
In order for us to locate sounds in the horizontal plane, we need a brain structure that can process information from both ears. Recall from the central auditory pathway that the first structure that processes information from both ears (binaural) is the superior olive.
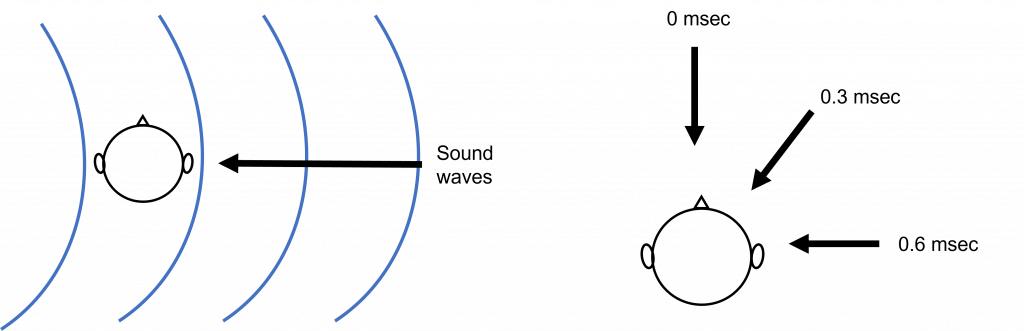
One of the primary functions of the superior olive is to help us figure out if a sound originates from the left or the right side of our head by determining this interaural time difference. Let’s imagine a sound wave originating from the left. The sound will interact with the left ear before it interacts with the right ear. When the sound interacts with the left ear, it will activate the hair cells in the left cochlea, the left spiral ganglion, and then the left cochlear nucleus (all monaural structures). The left cochlear nucleus cells will generate action potential that will travel along their axons toward the superior olive.
After a short delay, the sound wave will interact with the right ear, thus activating the hair cells of the right cochlea, the right spiral ganglion, and the right cochlear nucleus. The right cochlear nucleus will then generate its own action potential that will travel toward the superior olive. While this is occurring, the action potential generated by the left cochlear nucleus has traveled further along the left cochlear nucleus cell axon.
The action potentials generated from both the left and right cochlear nucleus will converge within the superior olive (a binaural structure). The axons of the cochlear nucleus cells have different length paths before they reach the neurons of the superior olive, allowing them to act as ‘delay lines’. The neurons of the superior olive act as coincidence detectors. When the action potentials arrive at one superior olive neuron at the same time, the postsynaptic potentials will summate, leading to maximal activation of this superior olive neuron and the generation of an action potential. If the action potentials do not arrive at the same time, then summation will not occur. The specific superior olive neuron that experiences the summation translates to where in the horizontal plane the sound originated, allowing us to localize sounds.
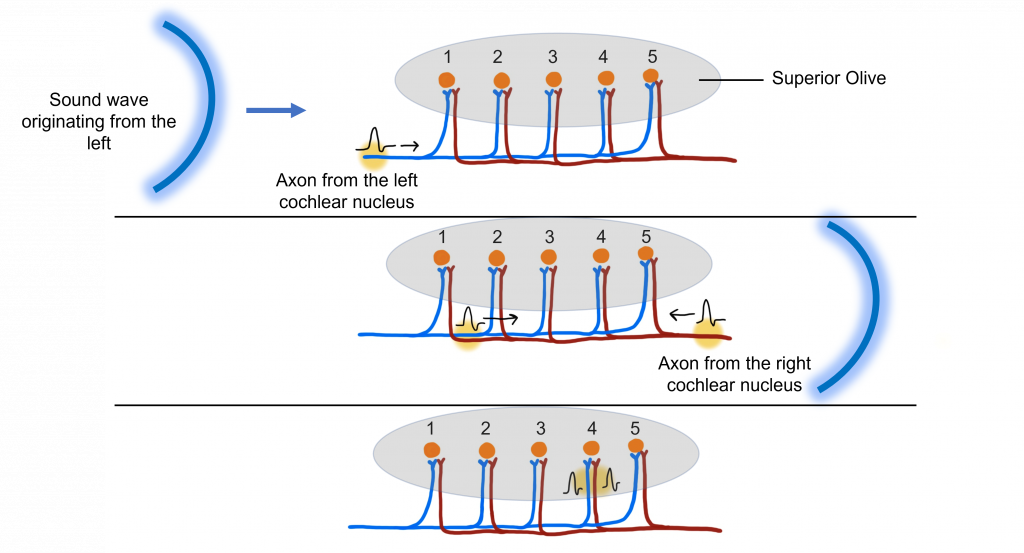
Humans are better at localizing sounds in the horizontal plane when we can detect the onset of the sound. However, when there are continuous tones, we need to utilize different ways to localize sounds in the horizontal plane. We can use interaural intensity (loudness) differences to localize sounds when they are continuous. Sounds are louder the closer you are to them. A difference in volume between what one ear and the other ear perceives can also be evaluated by neurons in the superior olive.
Interaural intensity differences are also useful in localizing high frequency sounds in the horizontal plane. At high frequencies (that have a wavelength shorter than the distance between our ears), the head creates a ‘sound shadow’ for the ear opposite the sound. When a high frequency sound is coming directly from the right side, the head creates a sound shadow for the left ear, making the sound louder for the right ear than it is for the left ear. Again, if a high frequency sound originates from directly in front of a person, then the sound will reach the ears with the same intensity, as the sound shadow will be behind the head. When a high frequency sound originates from an intermediate angle, then there will be intermediate intensity differences between the two ears.
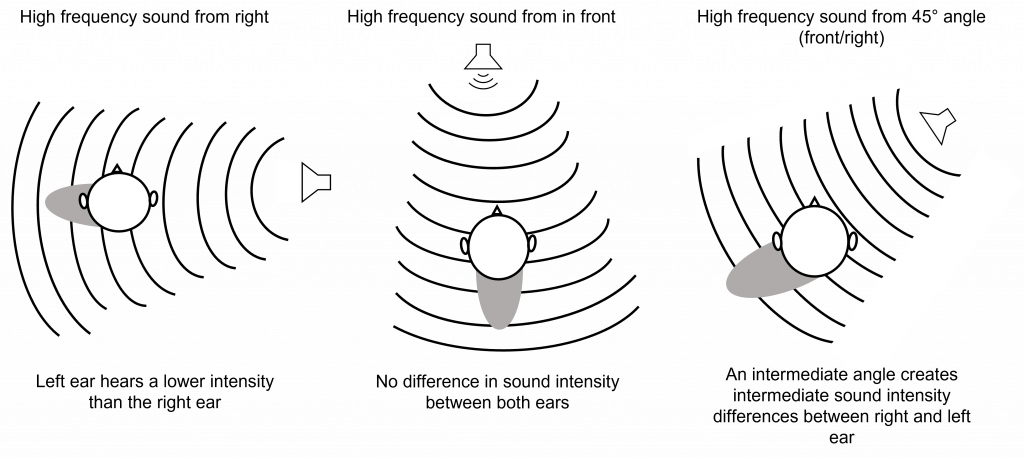
Low frequency sounds do not create a sound shadow like high frequency sounds. This is because the wavelength of low frequency waves (e.g. 200 Hz) have a wavelength longer than the width of the distance between the ears, so the intensity will be the same at both ears but the sound will arrive at the two ears at different times (interaural time difference).
High frequency sounds (e.g. 7000 Hz) have a wavelength that is shorter than the width of the distance between the ears, which is why the head creates a sound shadow that will alter the intensity of the sound between the two ears.
Interaural time difference is used for low frequency sounds in the 20-2000 Hz range, whereas interaural intensity difference is used for high frequency sounds in the 2000-20,000 Hz range. Together these two processes are called the duplex theory of sound localization.
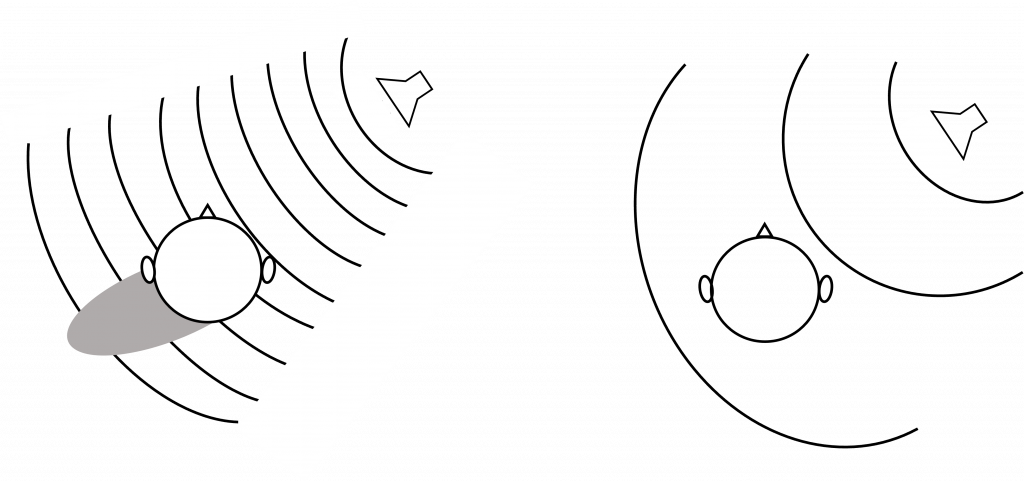
Localizing Sound in the Vertical Plane
Because both ears (in humans and most non-humans) are at the same height, sounds in the vertical plane that change in elevation alone can be difficult to localize. This is because the interaural time difference and interaural intensity between the two ears remains constant as elevation changes. This is why sometimes people and animals tilt their heads to try to hear better.
The structure of our pinna is important in vertical localization. Recall that our pinna is the external portion of our ear that funnels sounds into the auditory canal. For sound localization, the pinna has many ridges and folds, which cause sound waves to be reflected off these bumps and take different paths on their way to the auditory canal. The pinna of humans is relatively fixed in location, but other animals (like dogs) have the ability to move their pinna to better localize sounds.
Interestingly, not all animals have ears that are located at the same height on their head. Some species of owls have ear openings at different heights on their heads, increasing their ability to localize the source of a sound on the vertical axis. By having ears at different heights, the owls are able to use interaural time differences in the vertical plane because the sound will reach their ears at different times.
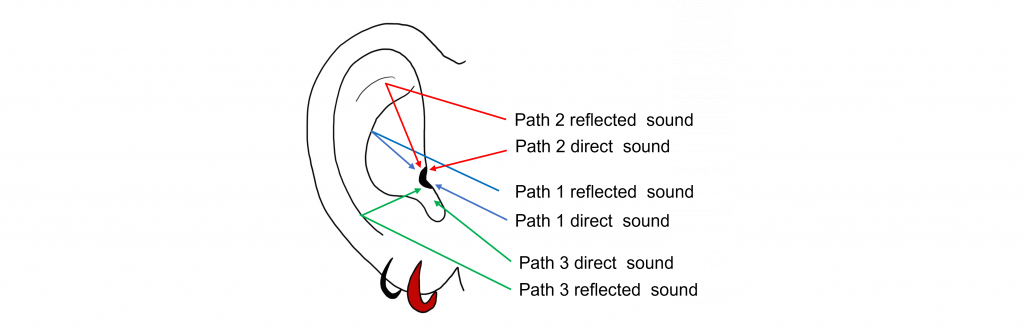
Key Takeaways
- Cranial nerve VIII carries auditory information into the cochlear nucleus, superior olive, inferior colliculus, medial geniculate nucleus of the thalamus, and finally to the primary auditory cortex.
- Structures of the central auditory pathway are tonotopically organized.
- In the horizontal plane, low frequency sounds are localized through interaural time differences and high frequency sounds are localized through interaural intensity differences.
- The superior olive is responsible for calculating interaural time differences.
- Sound localization in the vertical plane is more difficult for humans, but is aided by the structure of the pinna.
Test Yourself!
Attributions
Portions of this chapter were remixed and revised from the following sources:
- Open Neuroscience Initiative by Austin Lim. The original work is licensed under a Creative Commons Attribution-NonCommercial 4.0 International License.
Media Attributions
- Central Auditory Pathway © Jonathan E. Peelle is licensed under a CC BY (Attribution) license
- Tonotopic organization © Valerie Hedges is licensed under a CC BY-NC-SA (Attribution NonCommercial ShareAlike) license
- Auditory Cortex © Chittka L, Brockmann adapted by Valerie Hedges is licensed under a CC BY (Attribution) license
- Localizing sound © Valerie Hedges is licensed under a CC BY-NC-SA (Attribution NonCommercial ShareAlike) license
- Superior Olive © Valerie Hedges is licensed under a CC BY-NC-SA (Attribution NonCommercial ShareAlike) license
- Interaural intensity difference © Valerie Hedges is licensed under a CC BY-NC-SA (Attribution NonCommercial ShareAlike) license
- High and Low frequency sounds © Valerie Hedges is licensed under a CC BY-NC-SA (Attribution NonCommercial ShareAlike) license
- localizing sound in vertical plane © Valerie Hedges is licensed under a CC BY-NC-SA (Attribution NonCommercial ShareAlike) license
occurs on the same side of the body
denotes the side of the body opposite to the side where a structure started
Organized such that adjacent physical areas are responsible for conveying information from adjacent frequencies
The time between when sound reaches the first ear until the time it reaches the second ear
add together
Difference in volume experienced between the ears
funnel-shaped structure of the outer ear