Images of Animations
Chapter 1
Animation 1
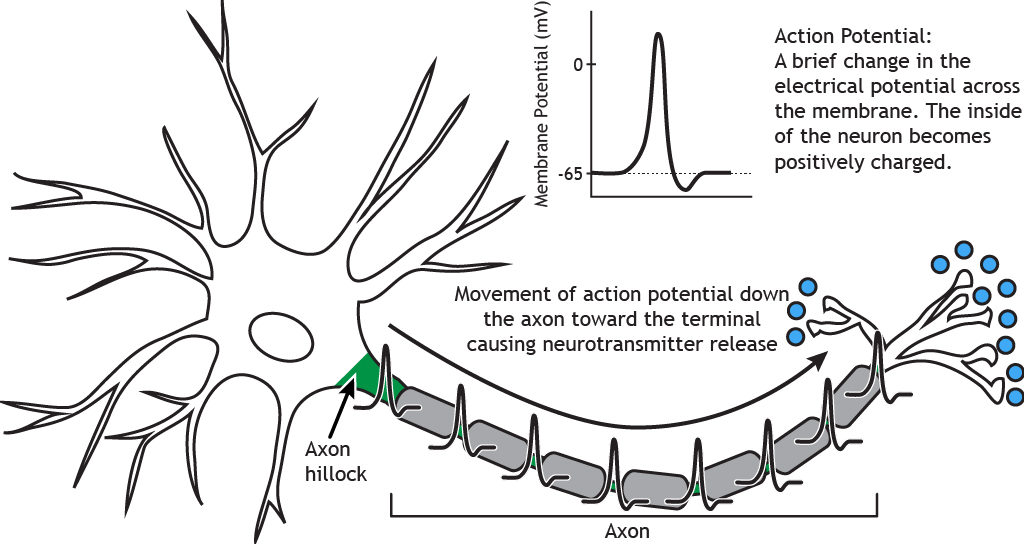
Chapter 2
Animation 1
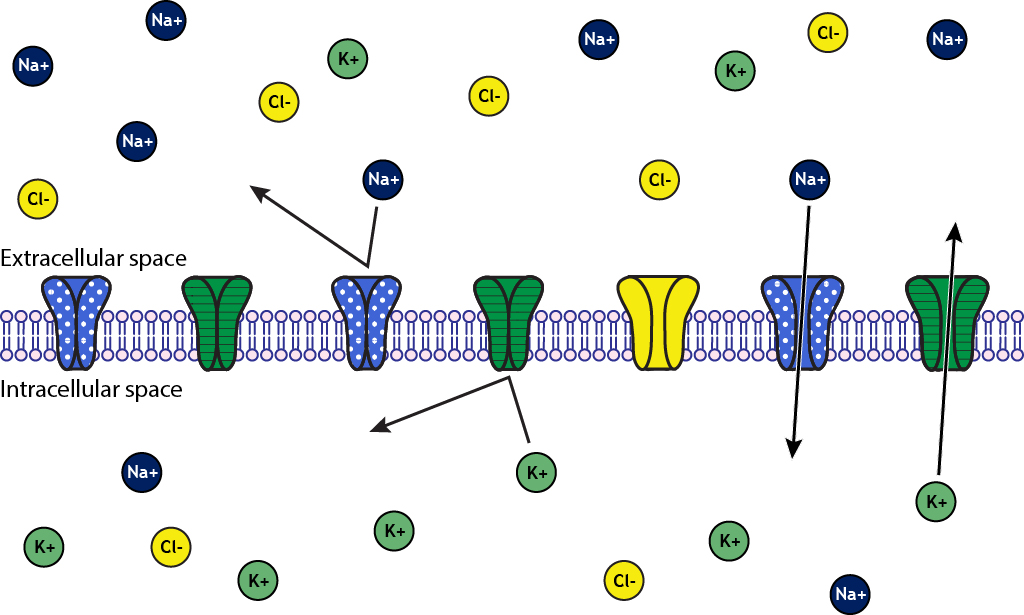
Animation 2

Animation 3
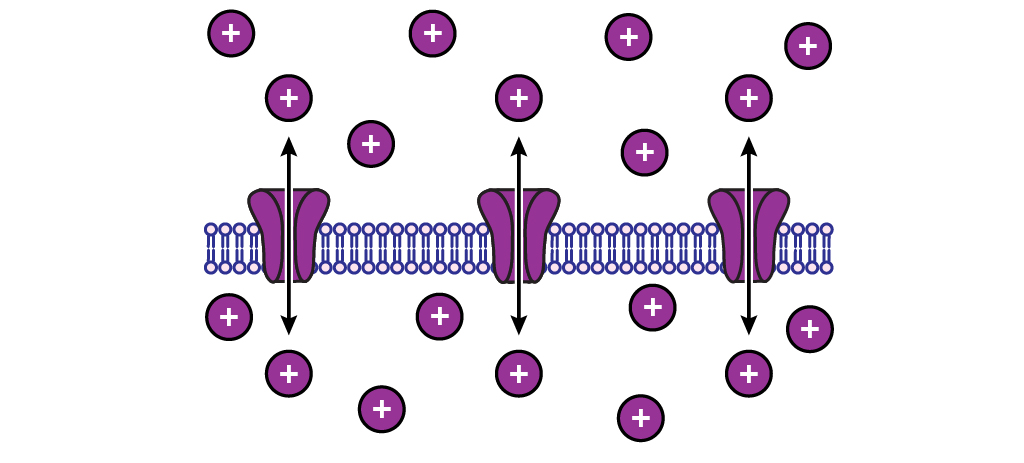
Chapter 3
Animation 1
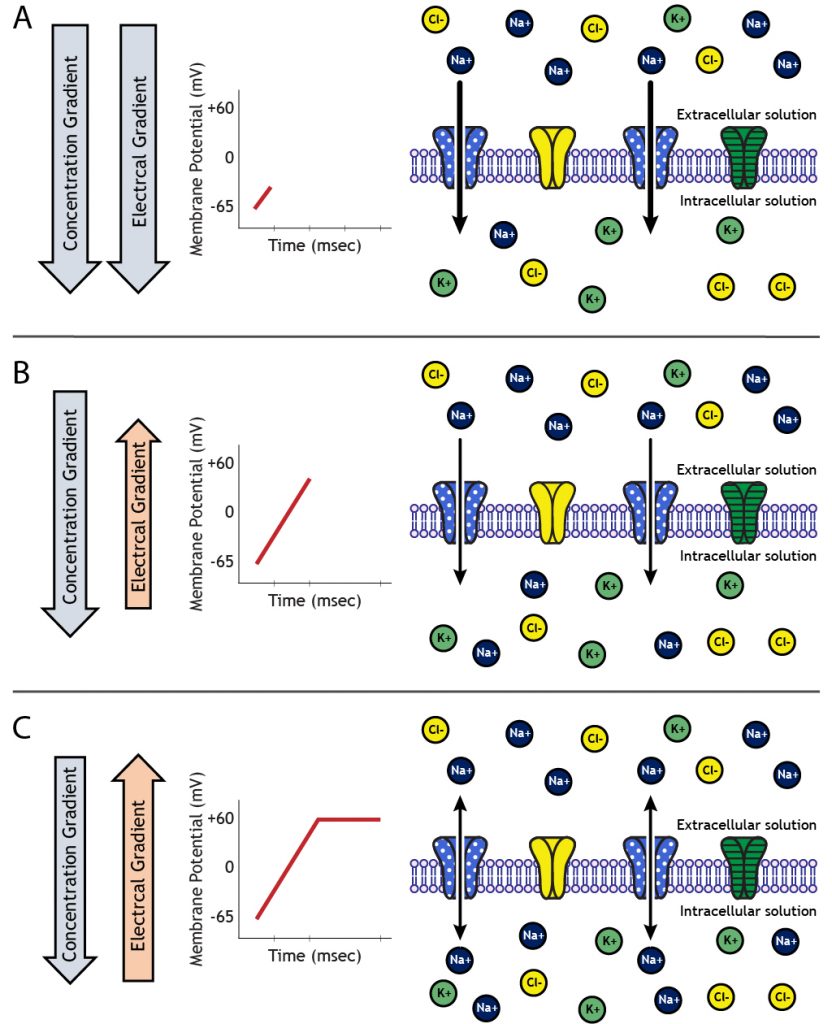
Chapter 4
Animation 1
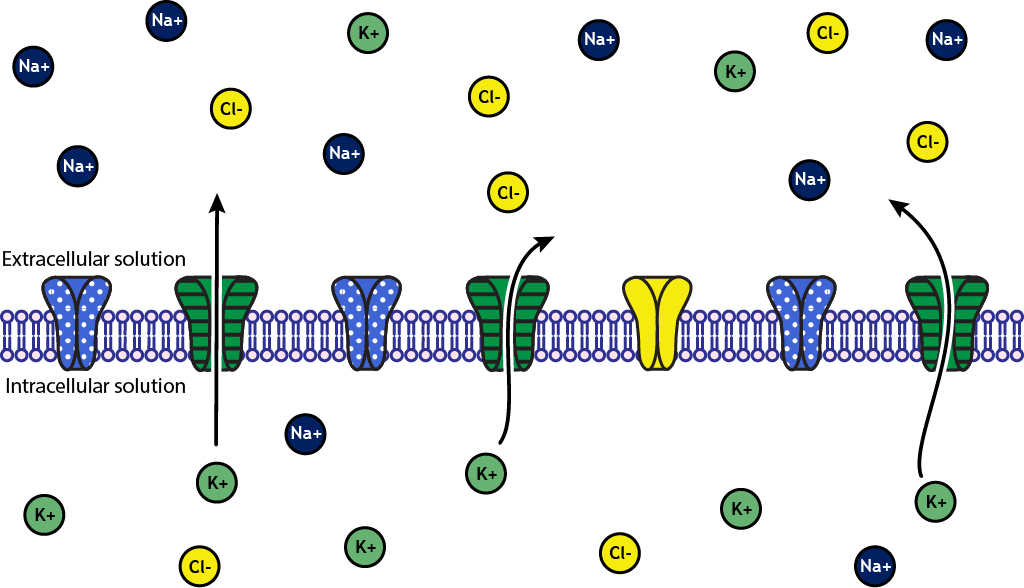
Animation 2

Animation 3
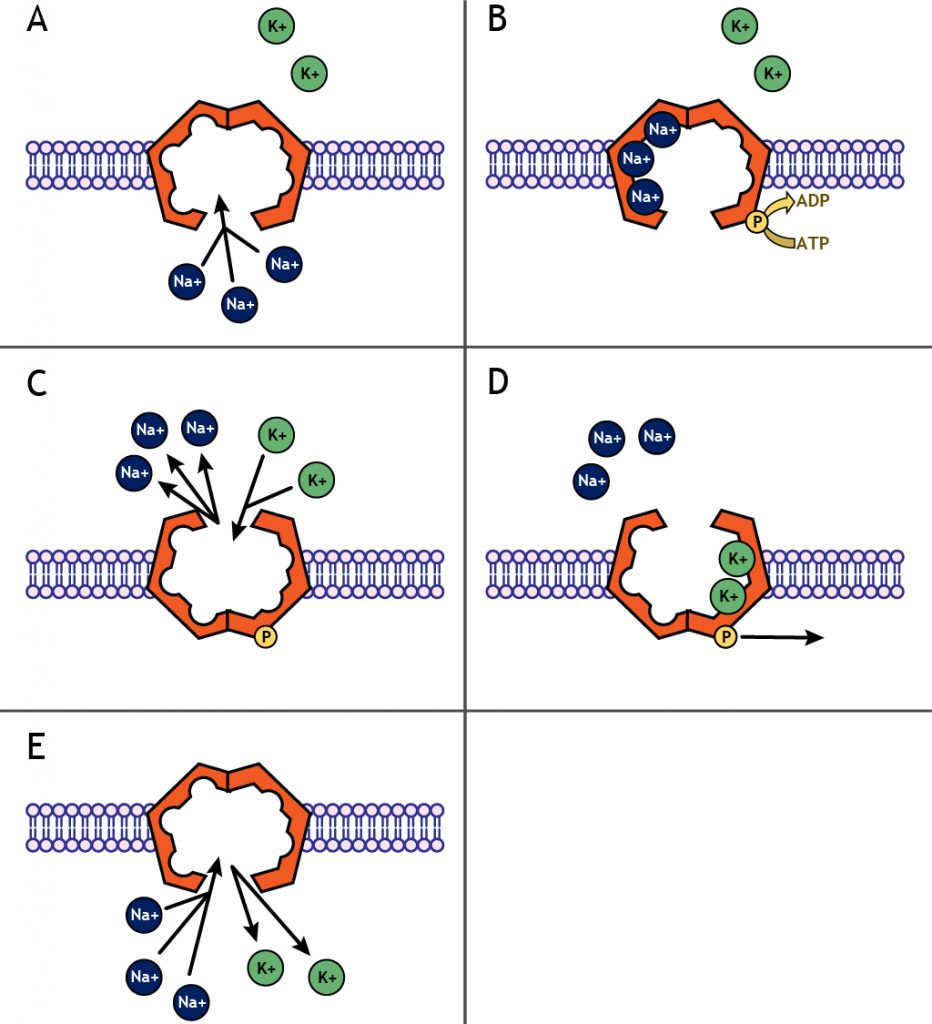
Chapter 5
Animation 1
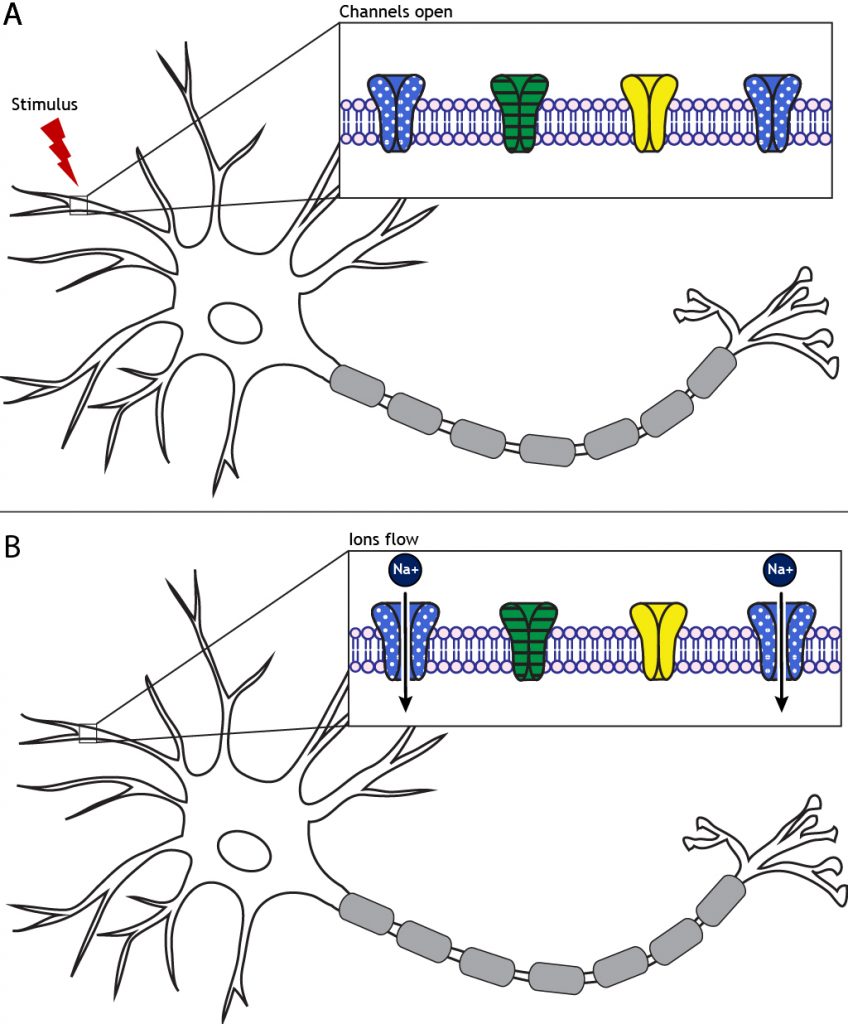
Animation 2

Animation 3
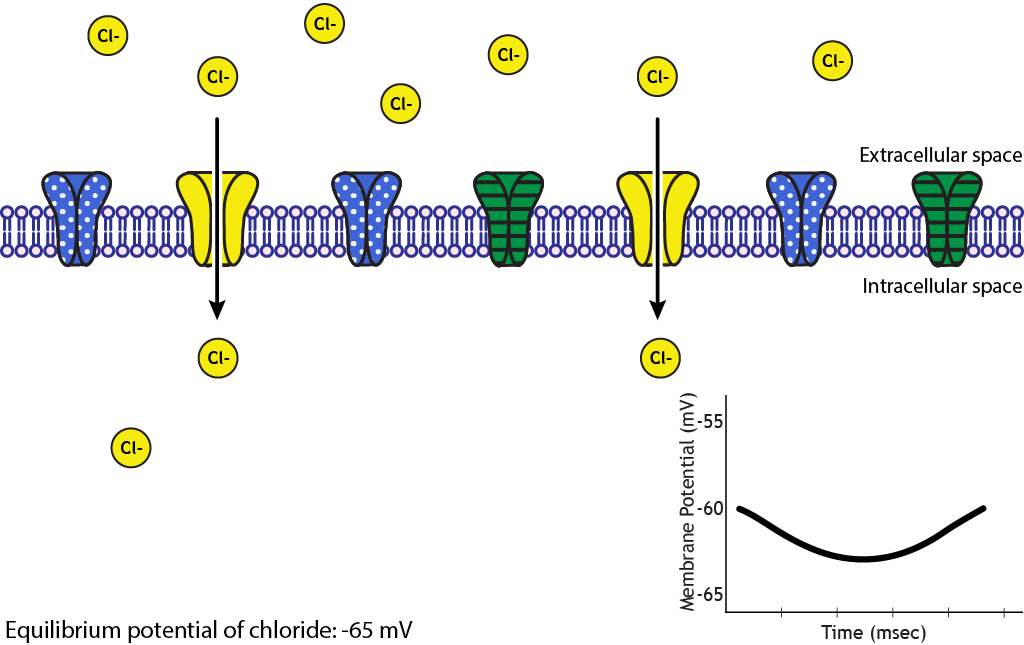
Animation 4
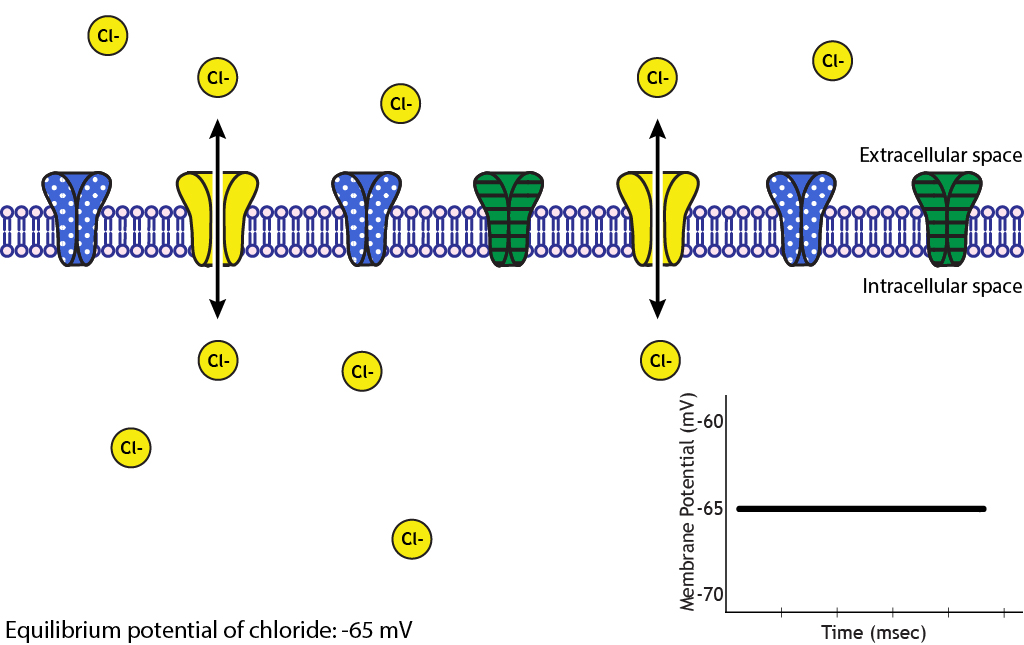
Animation 5
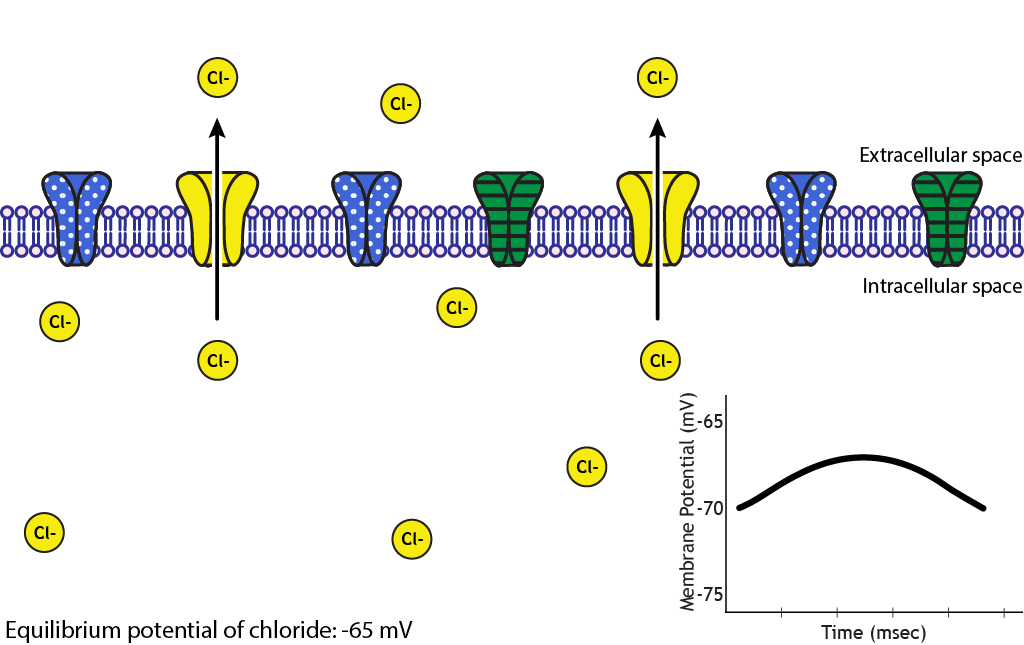
Animation 6
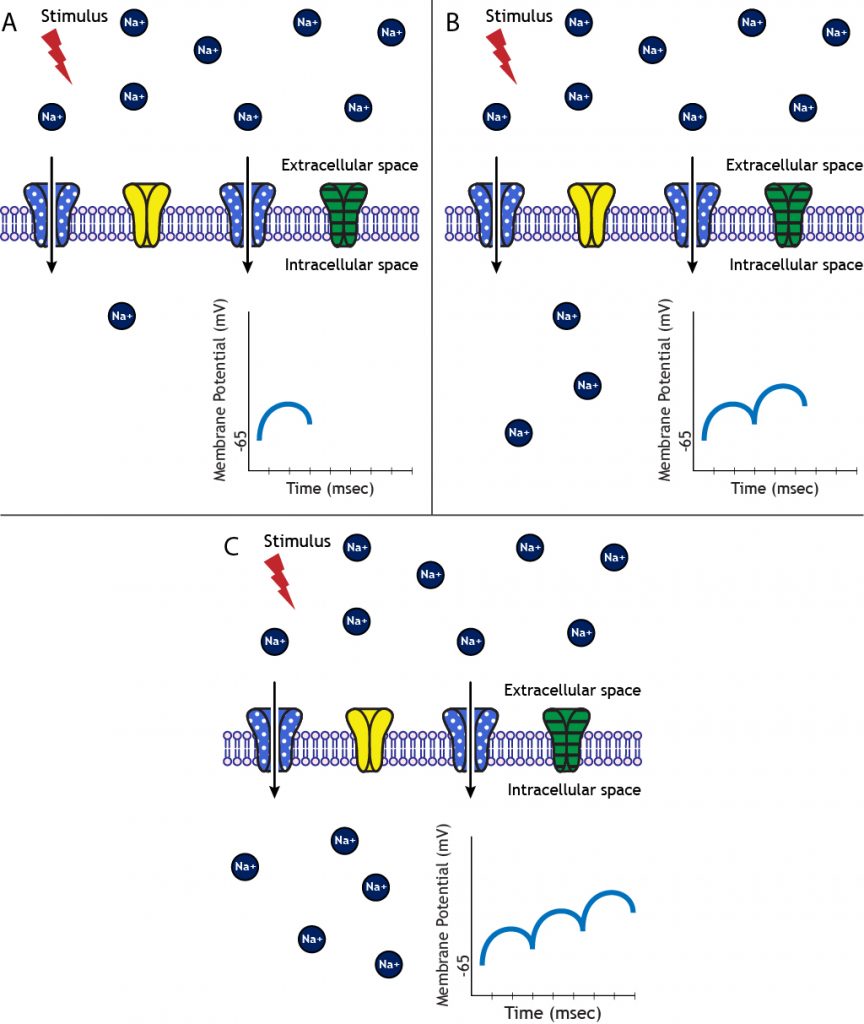
Animation 7
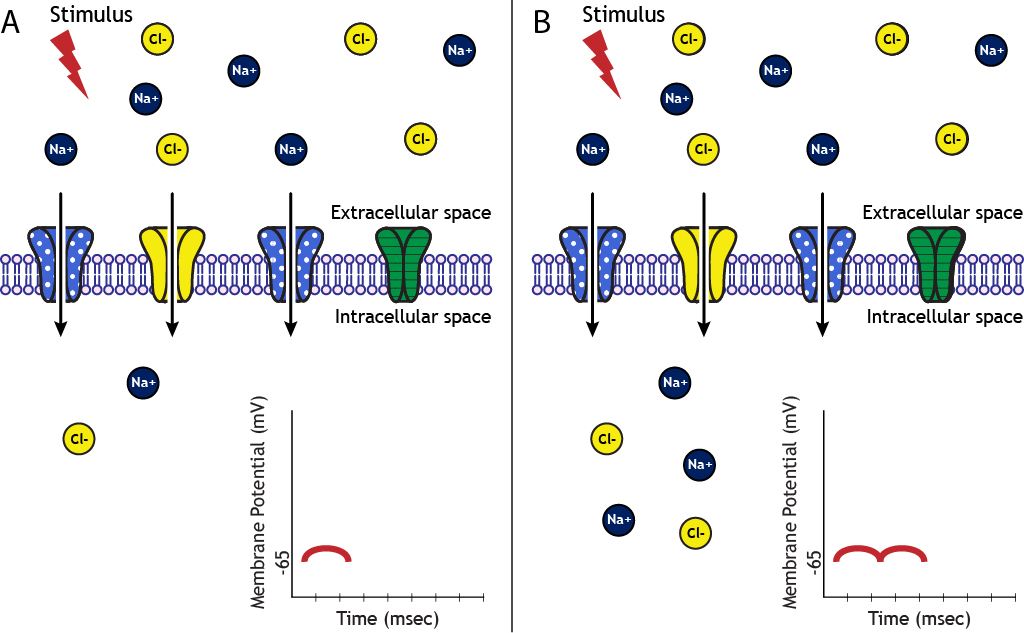
Chapter 6
Animation 1
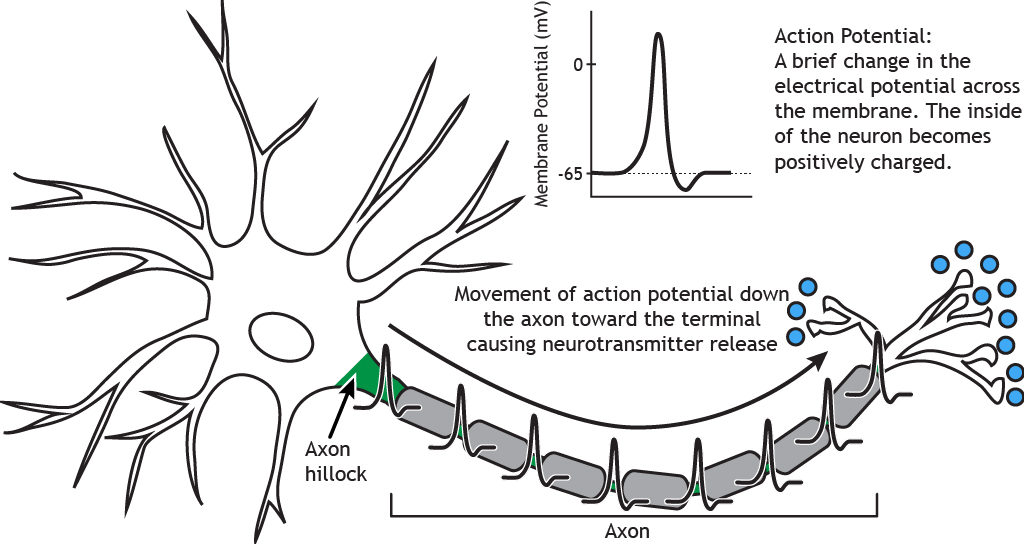
Animation 2
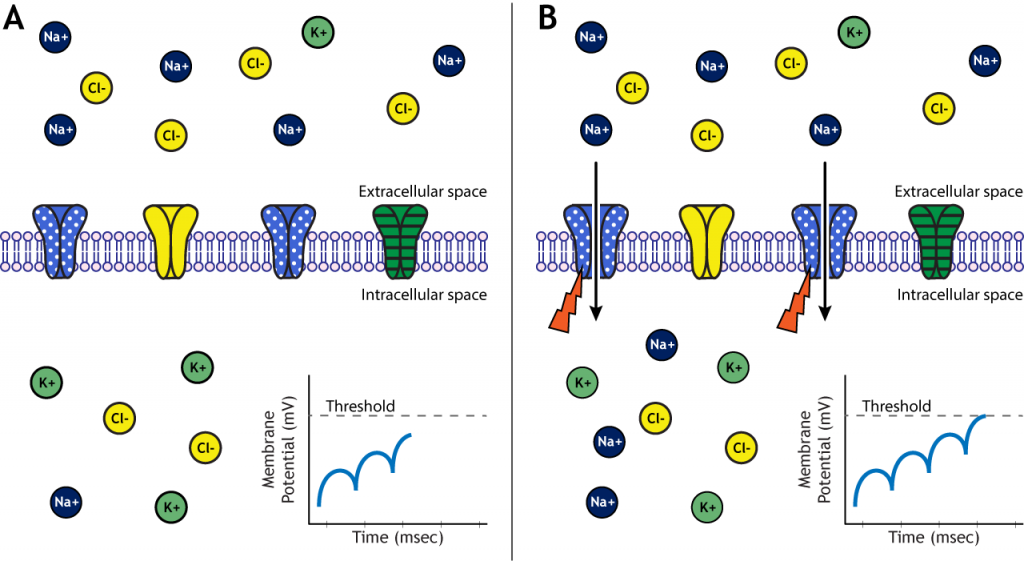
Animation 3
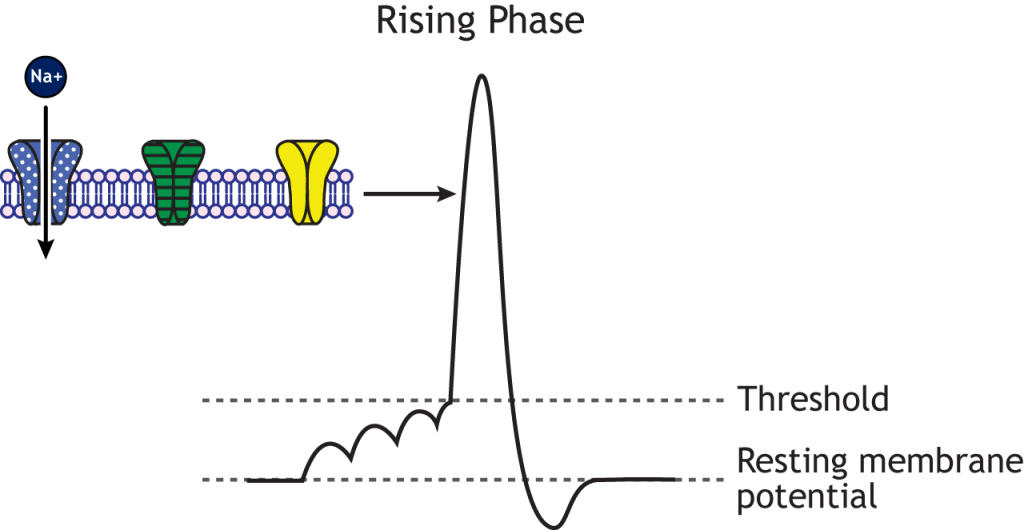
Animation 4
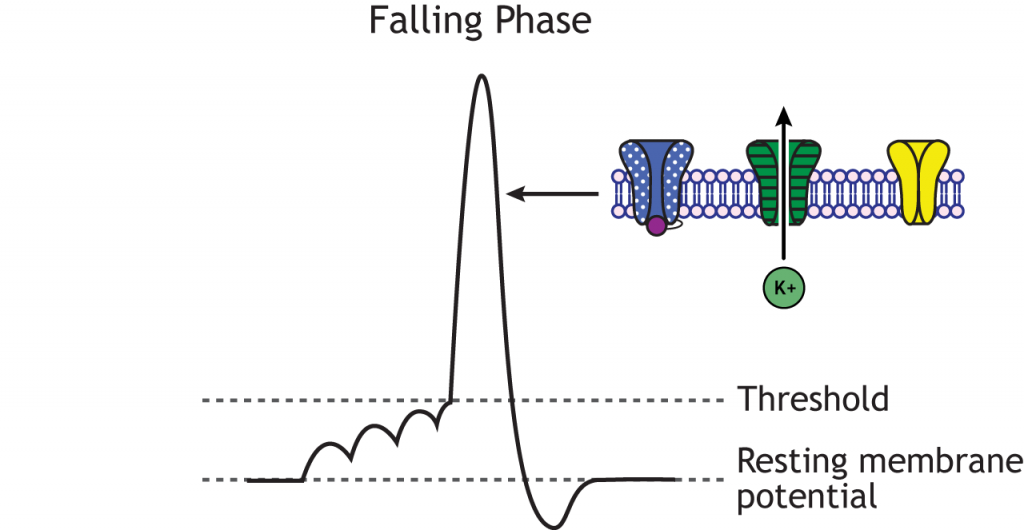
Animation 5
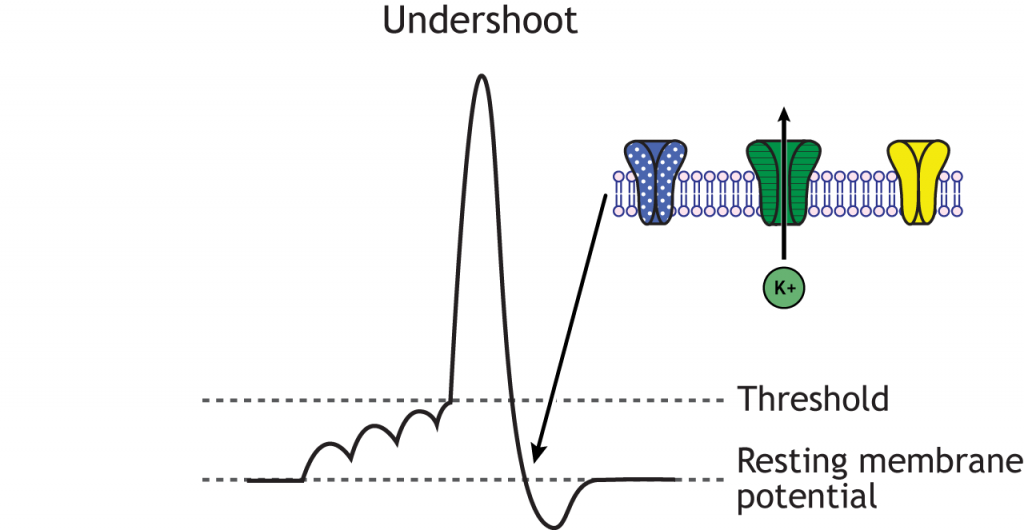
Animation 6
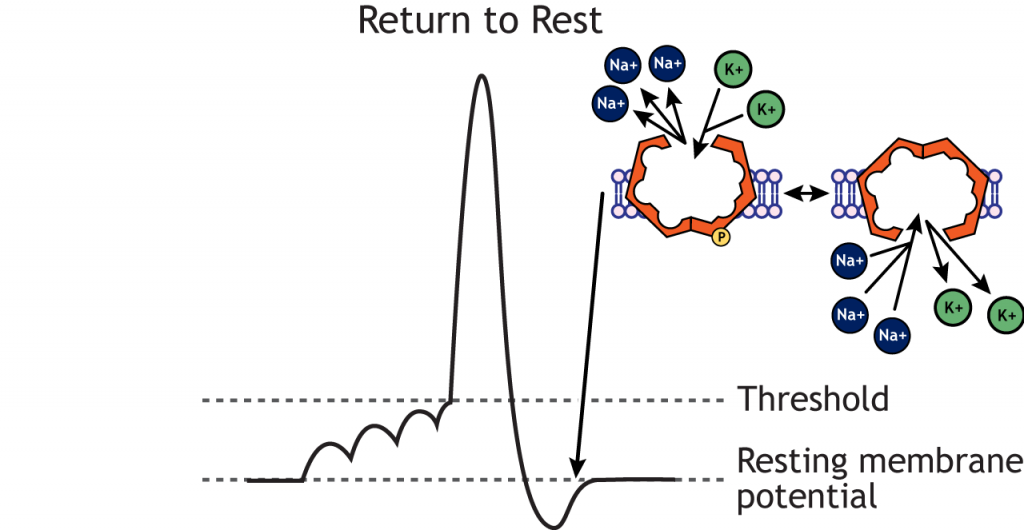
Animation 7
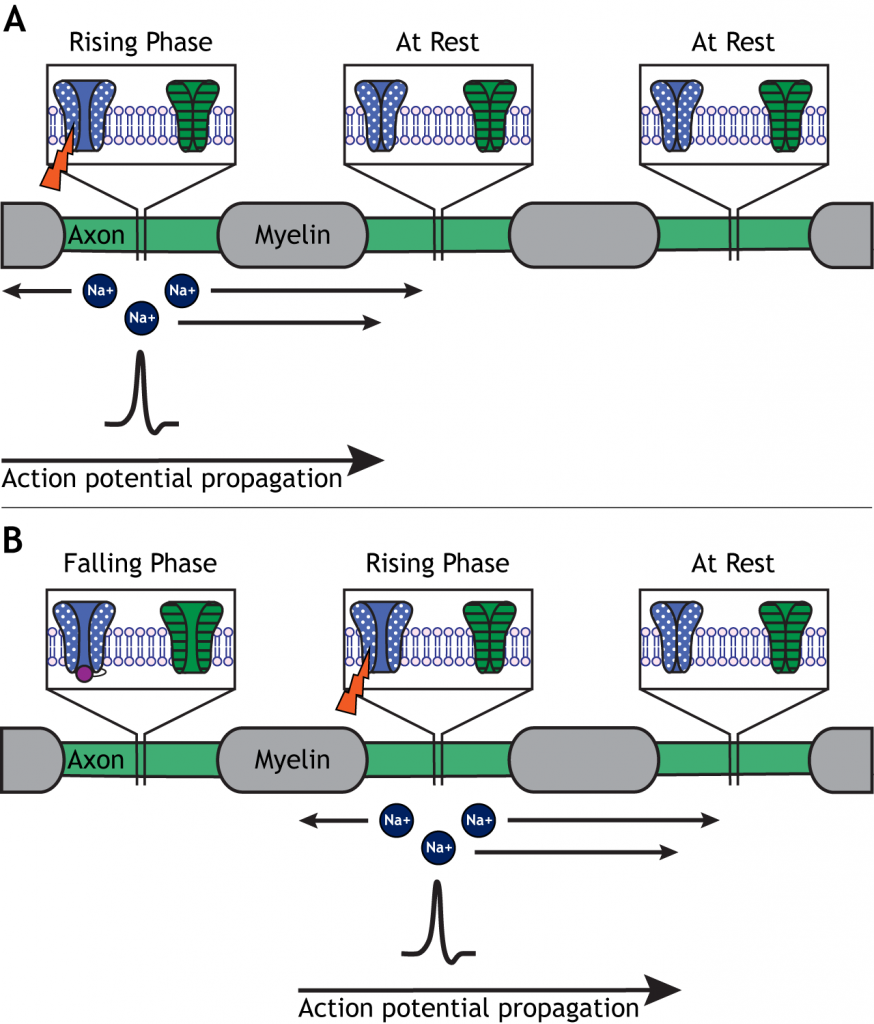
Animation 8
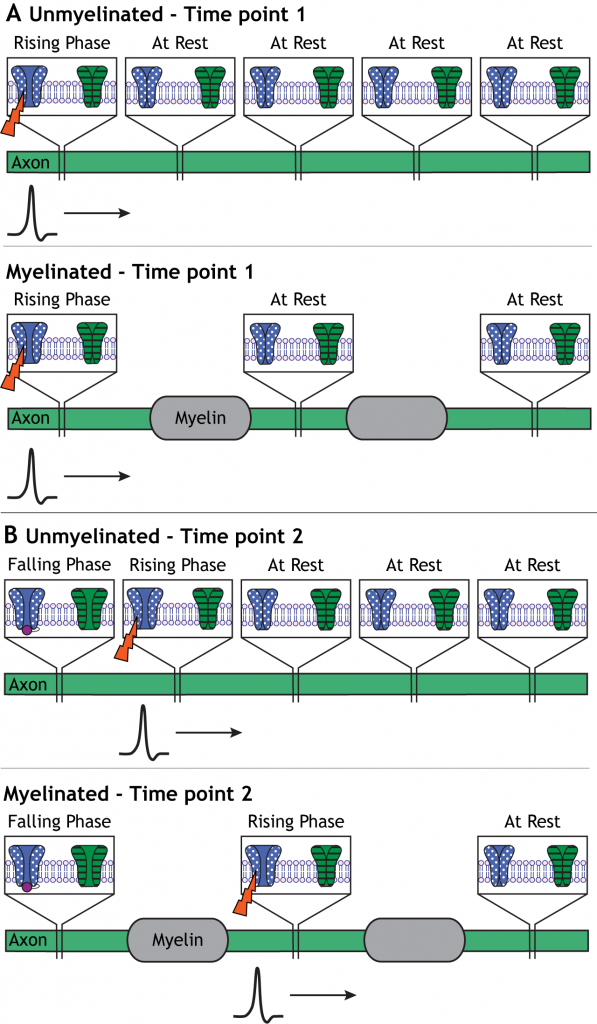
Chapter 7
Animation 1
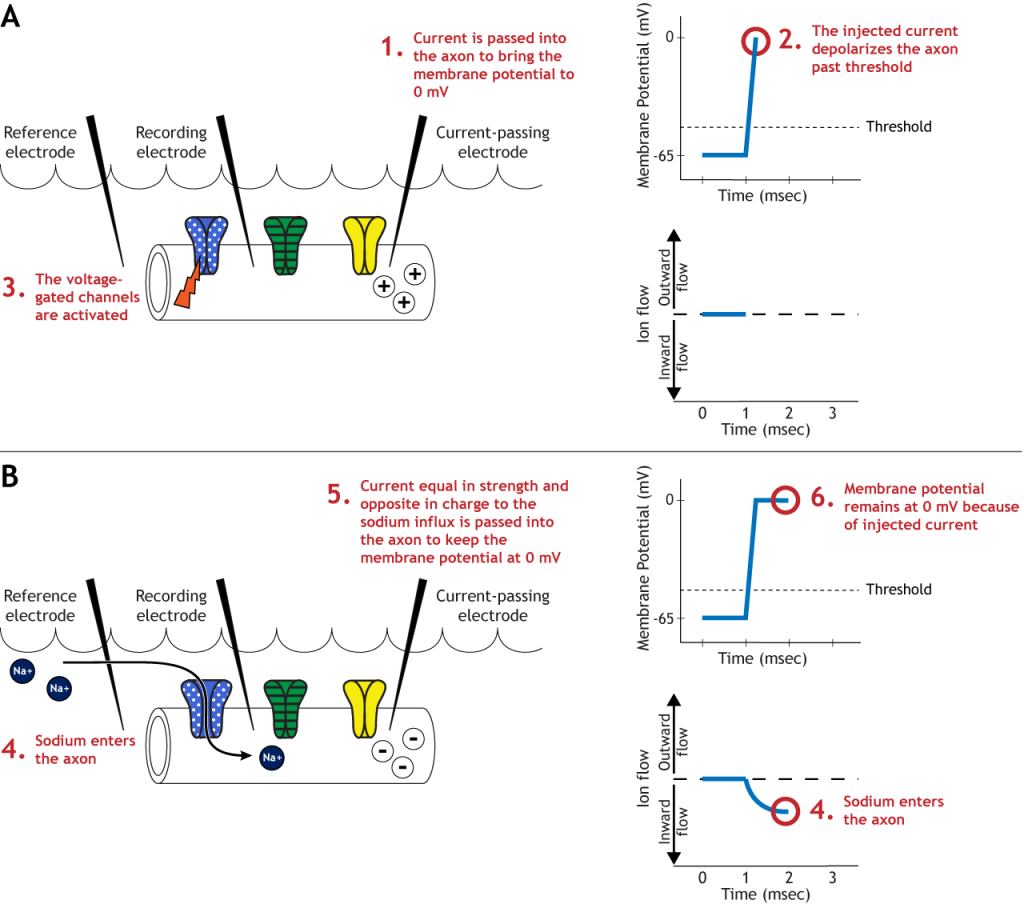
Animation 2
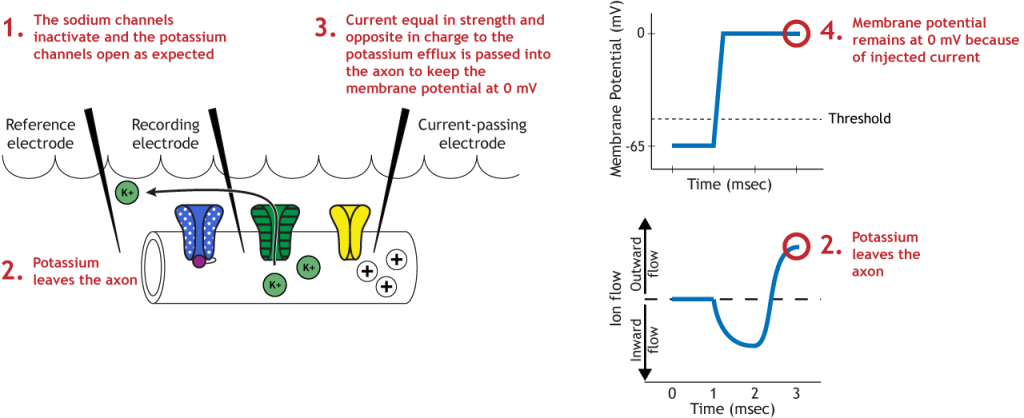
Chapter 8
Animation 1

Animation 2

Animation 3
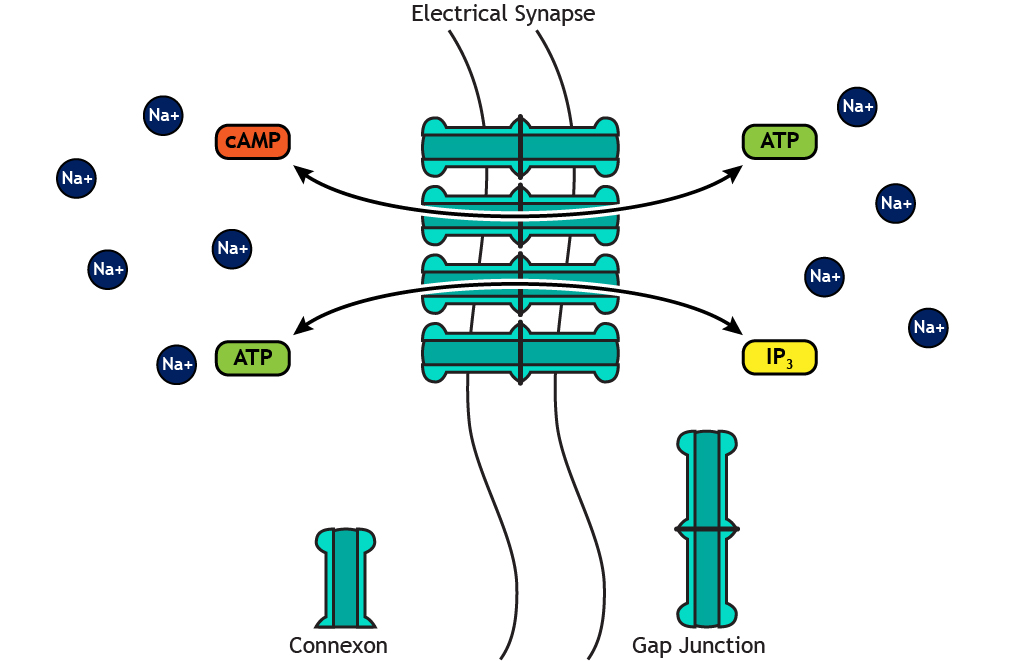
Animation 4
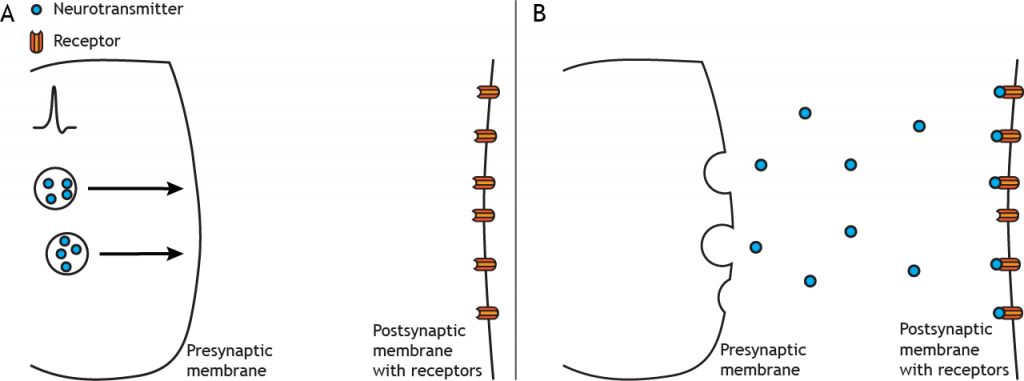
Chapter 10
Animation 1
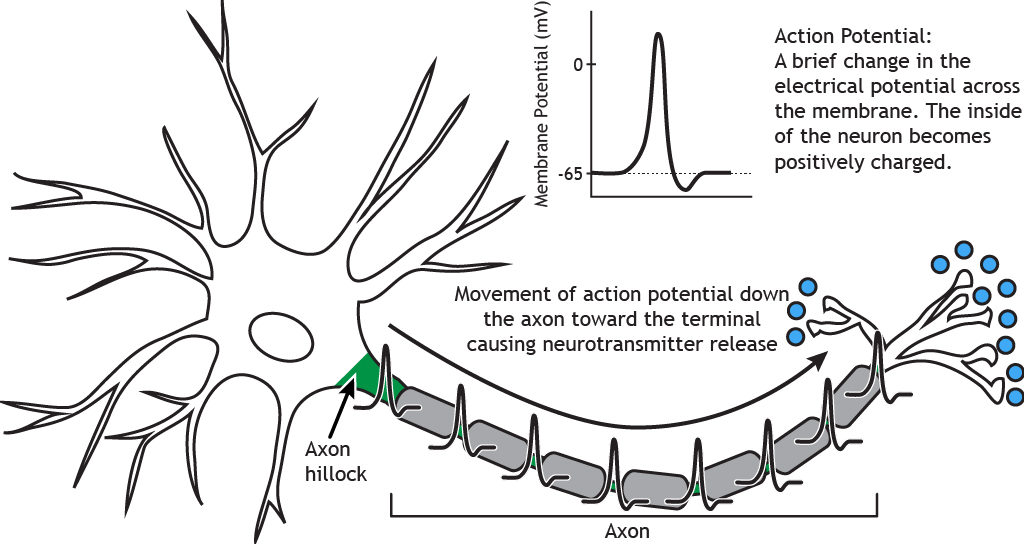
Animation 2
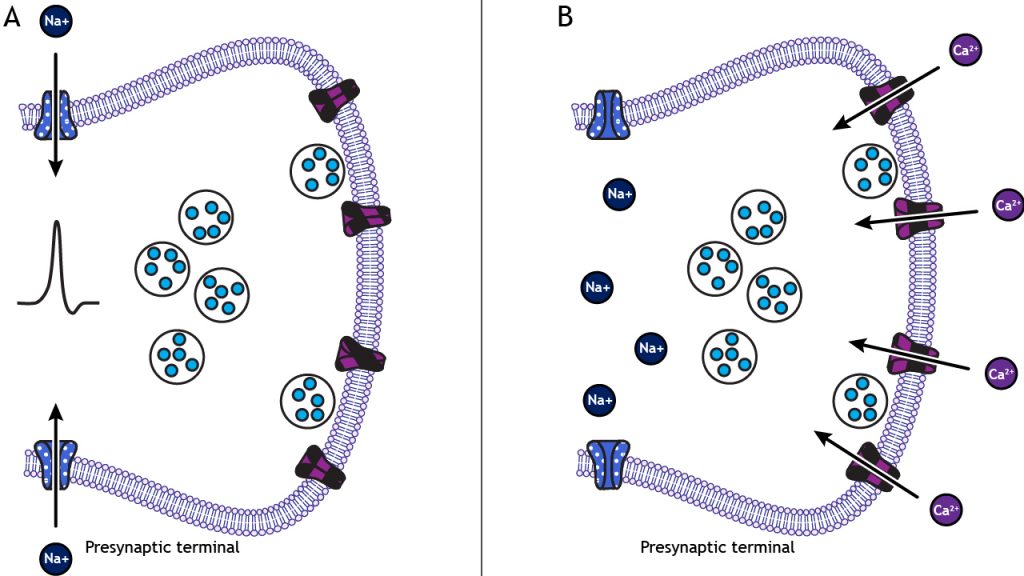
Animation 3
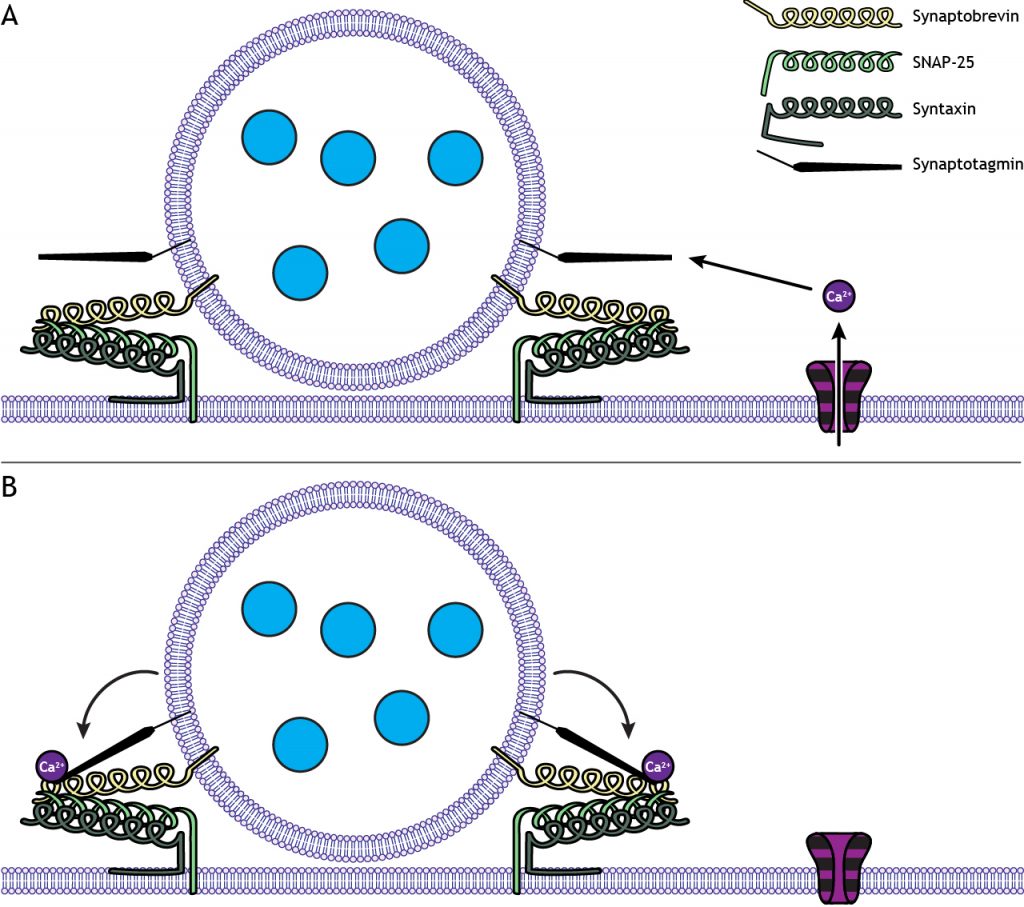
Animation 4
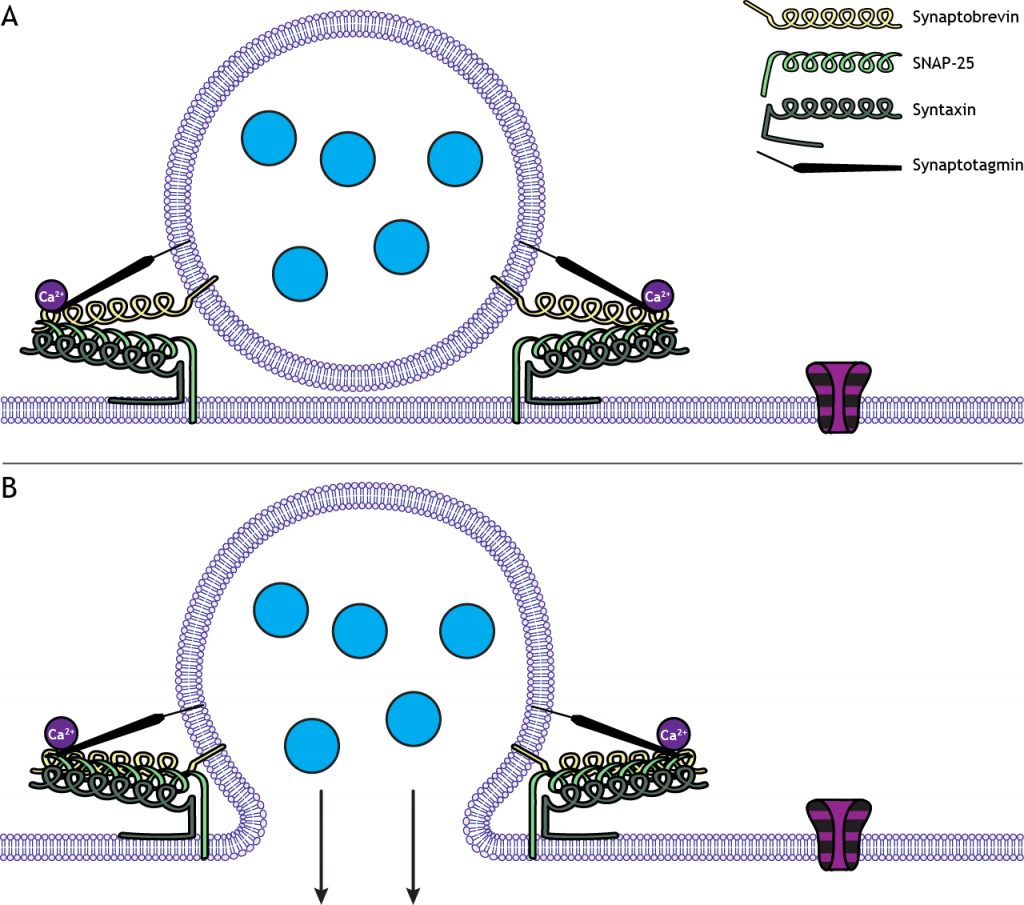
Chapter 11
Animation 1
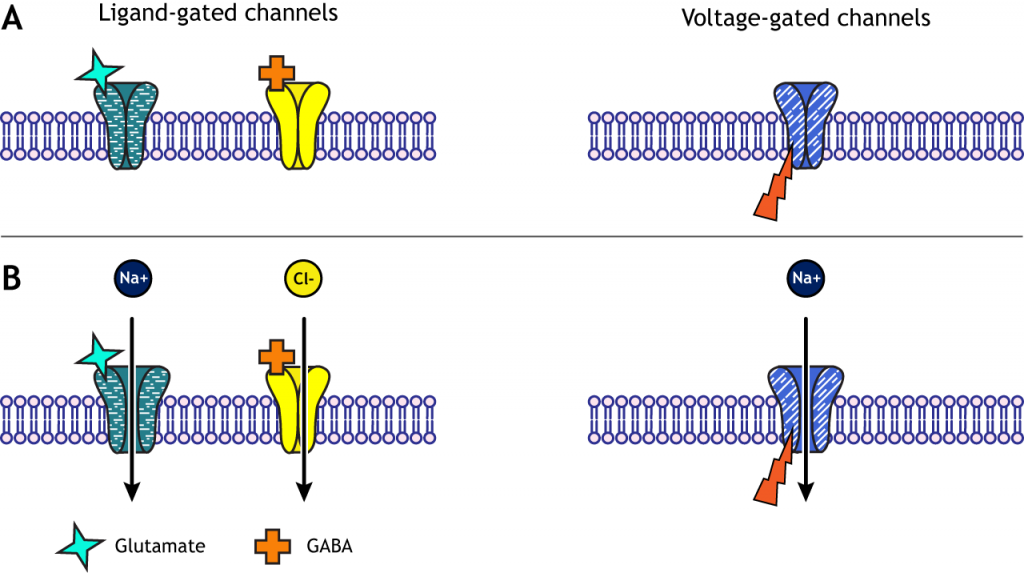
Animation 2
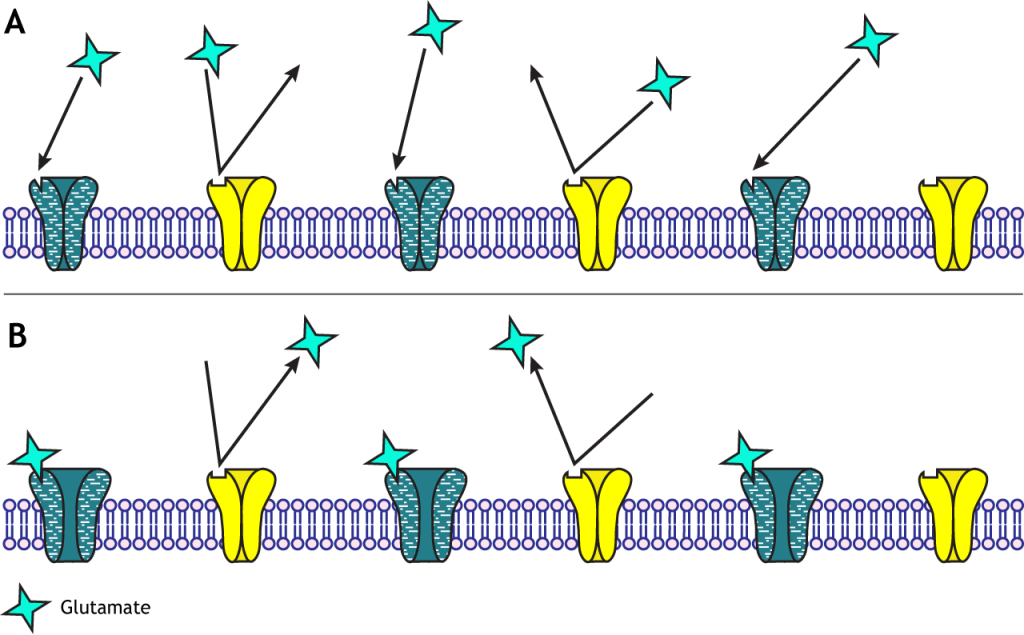
Animation 3
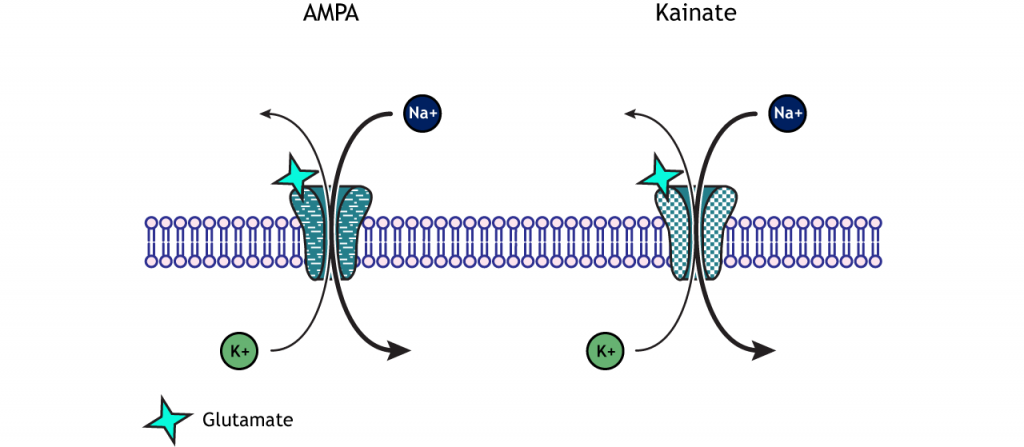
Animation 4
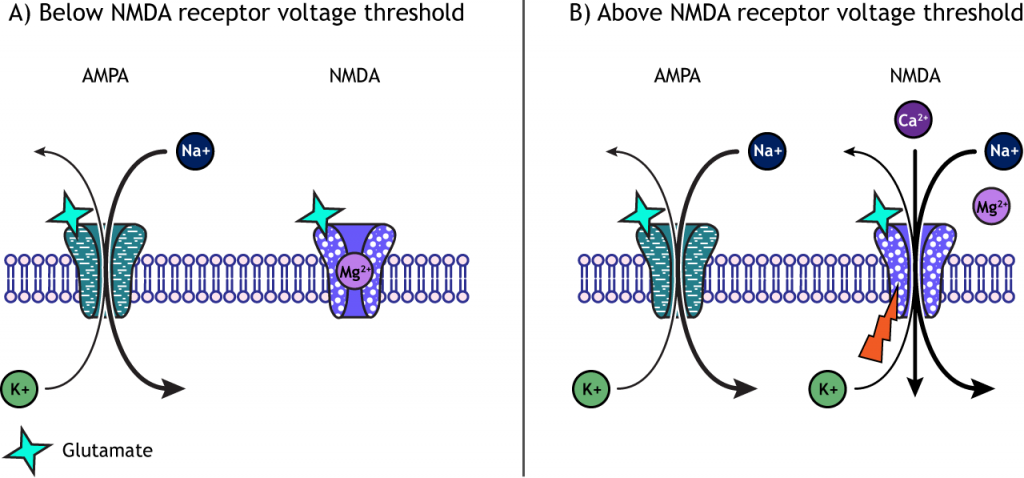
Animation 5
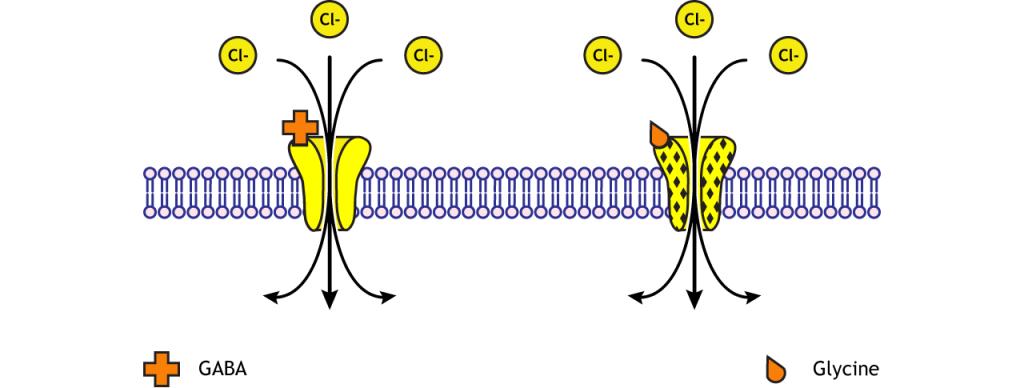
Animation 6
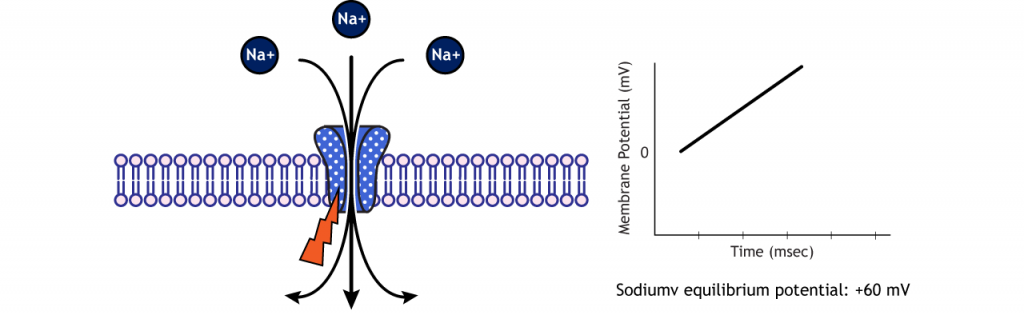
Animation 7
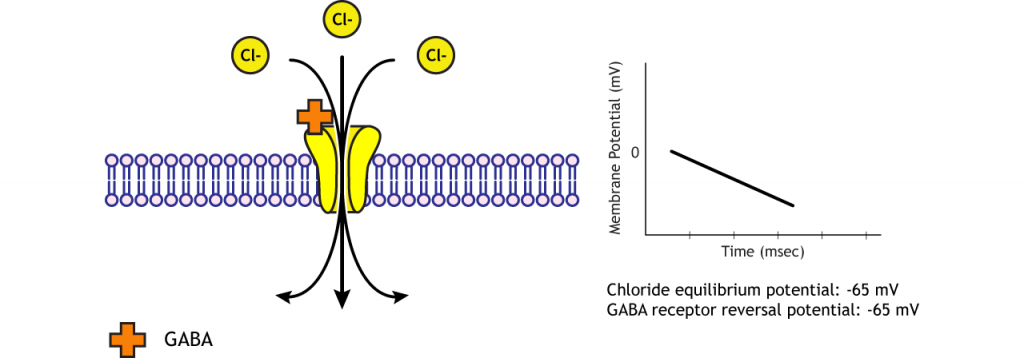
Animation 8
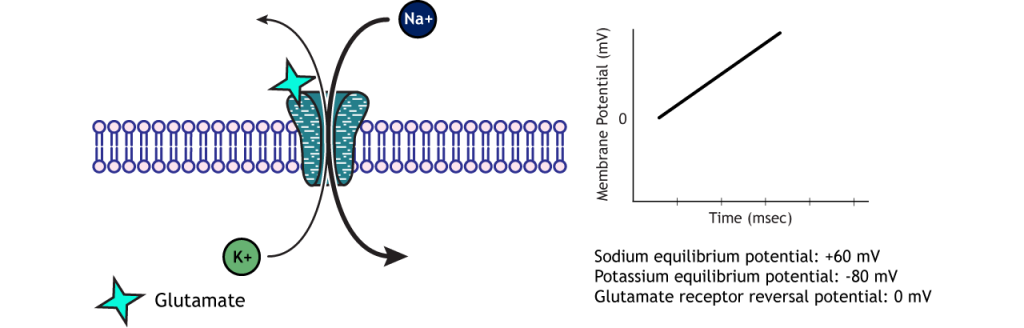
Animation 9
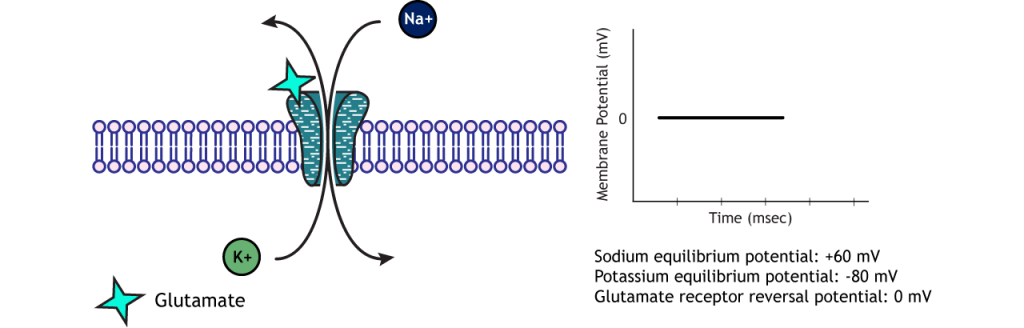
Chapter 12
Animation 1
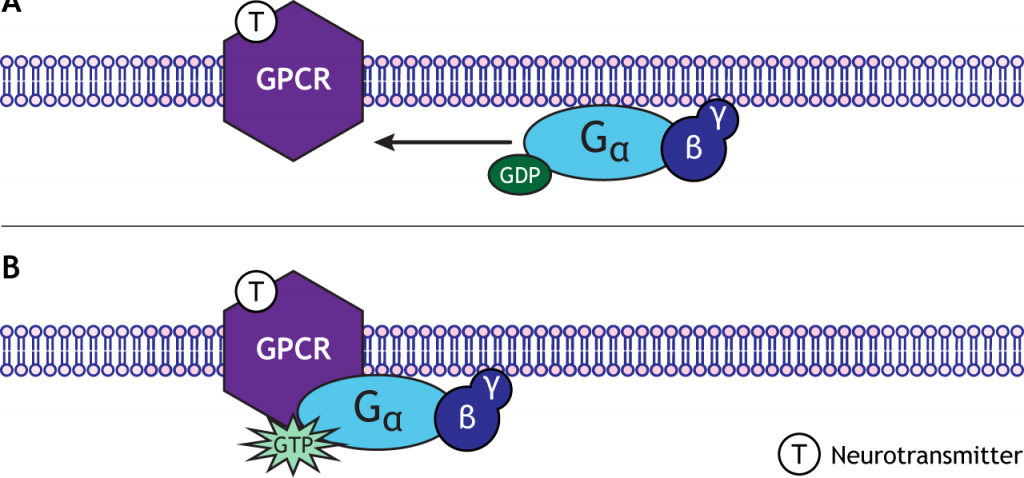
Animation 2
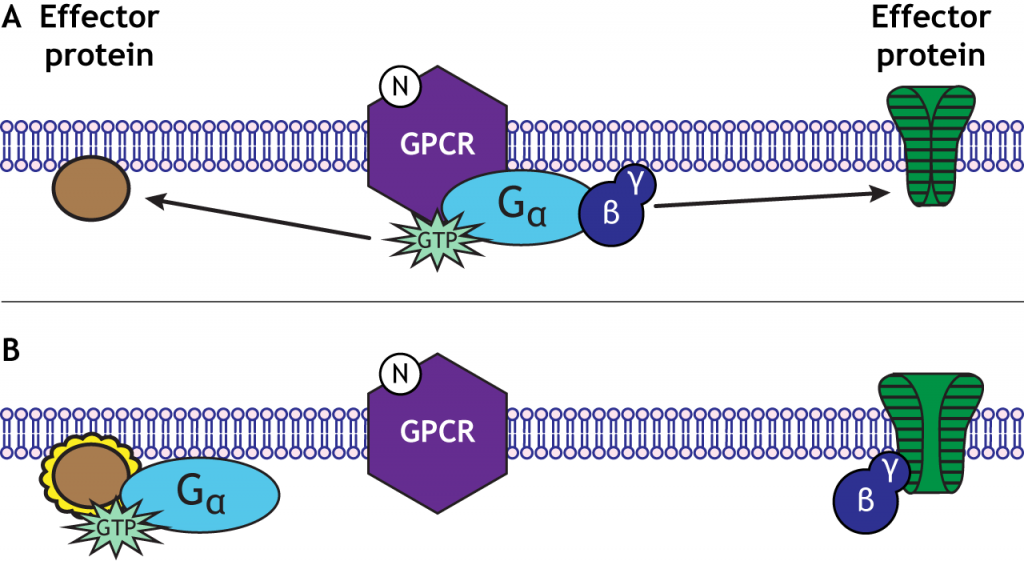
Animation 3
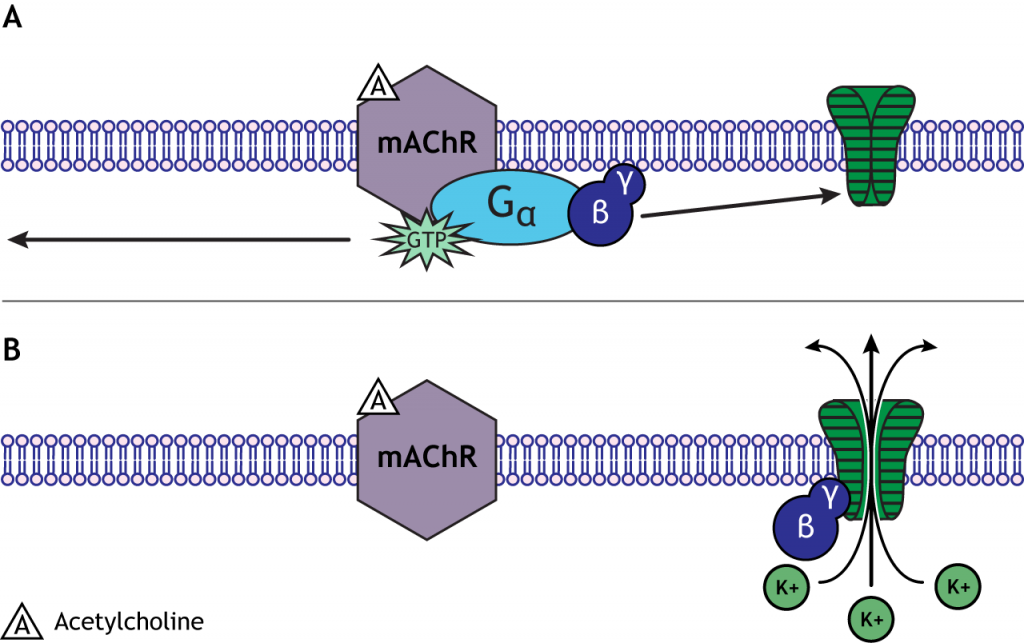
Animation 4
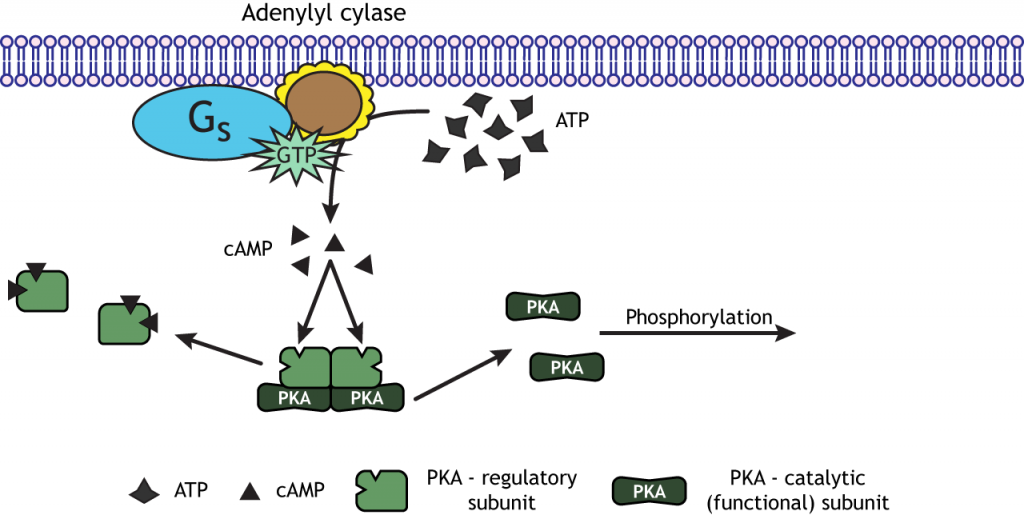
Animation 5
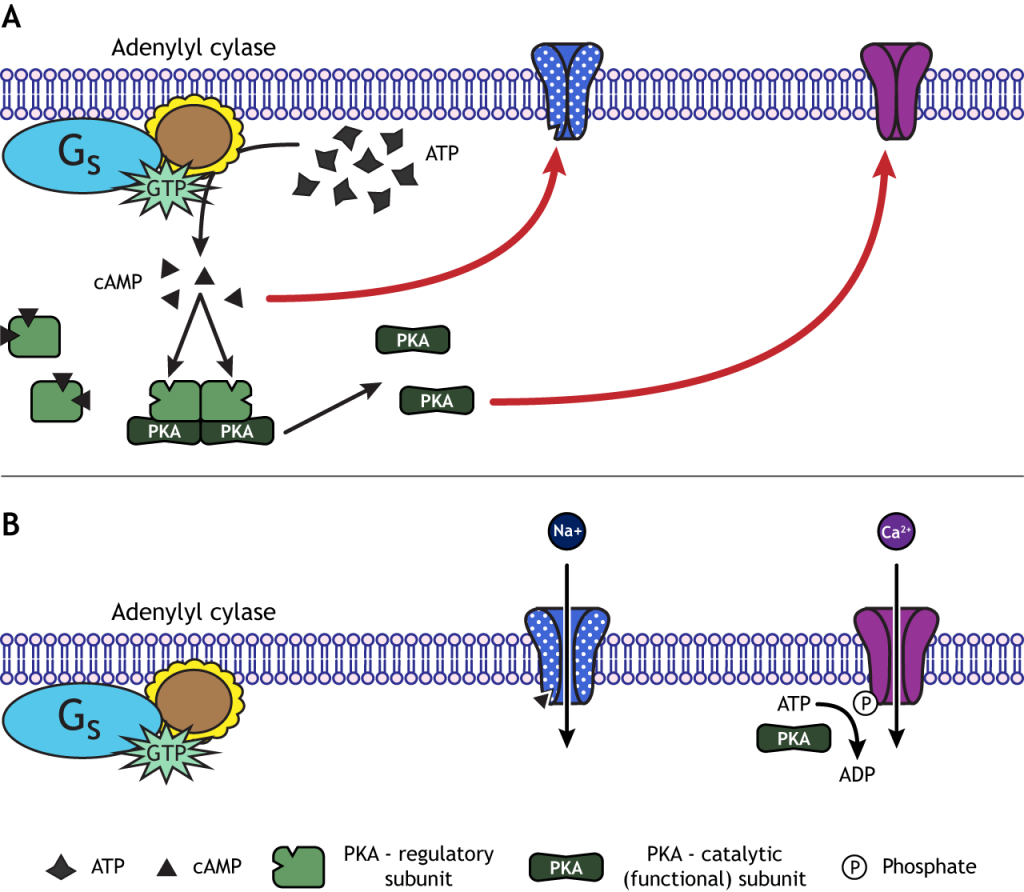
Animation 6
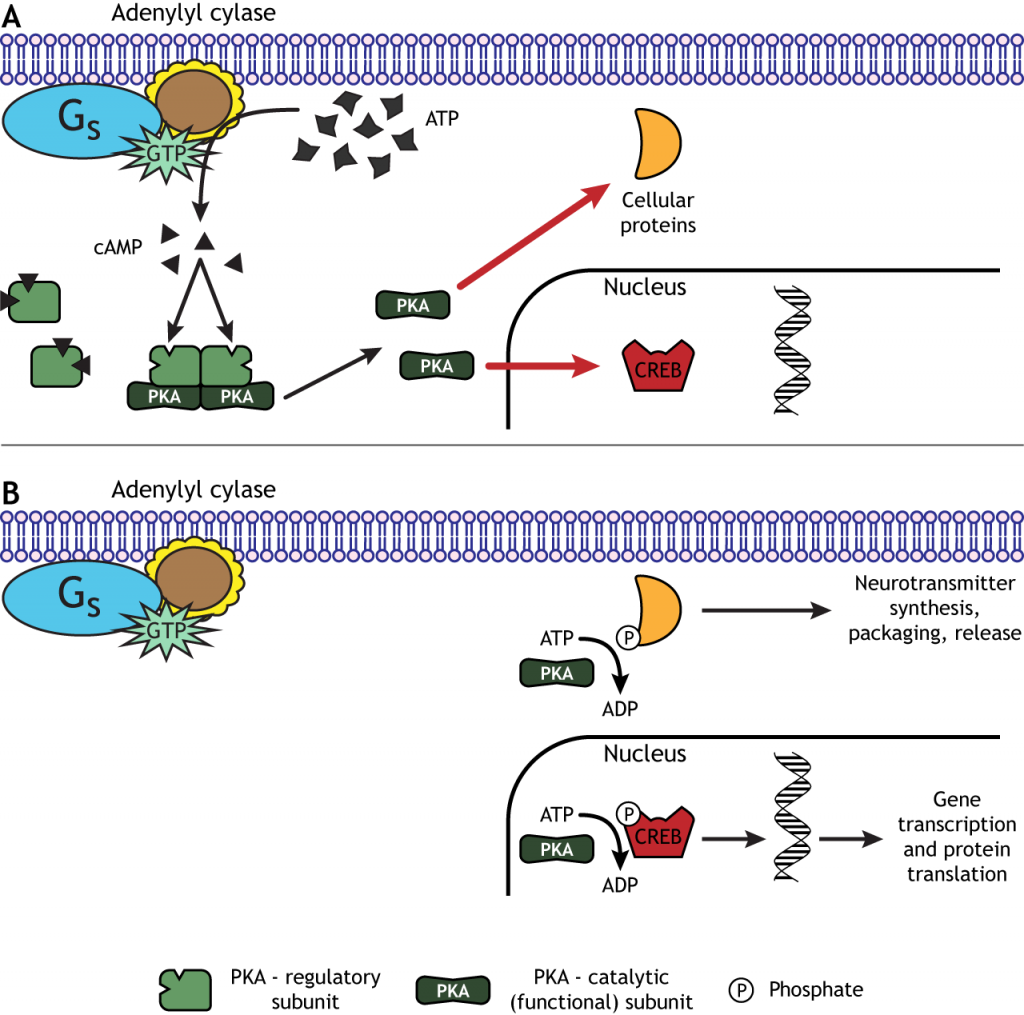
Animation 7
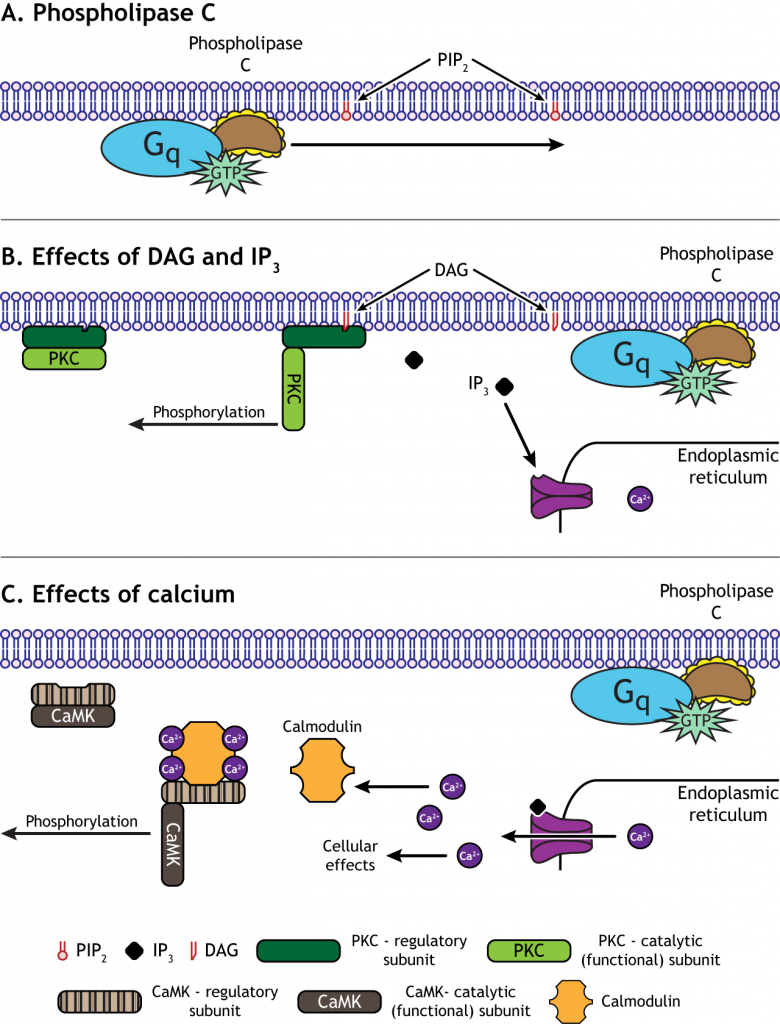
Chapter 19
Animation 1
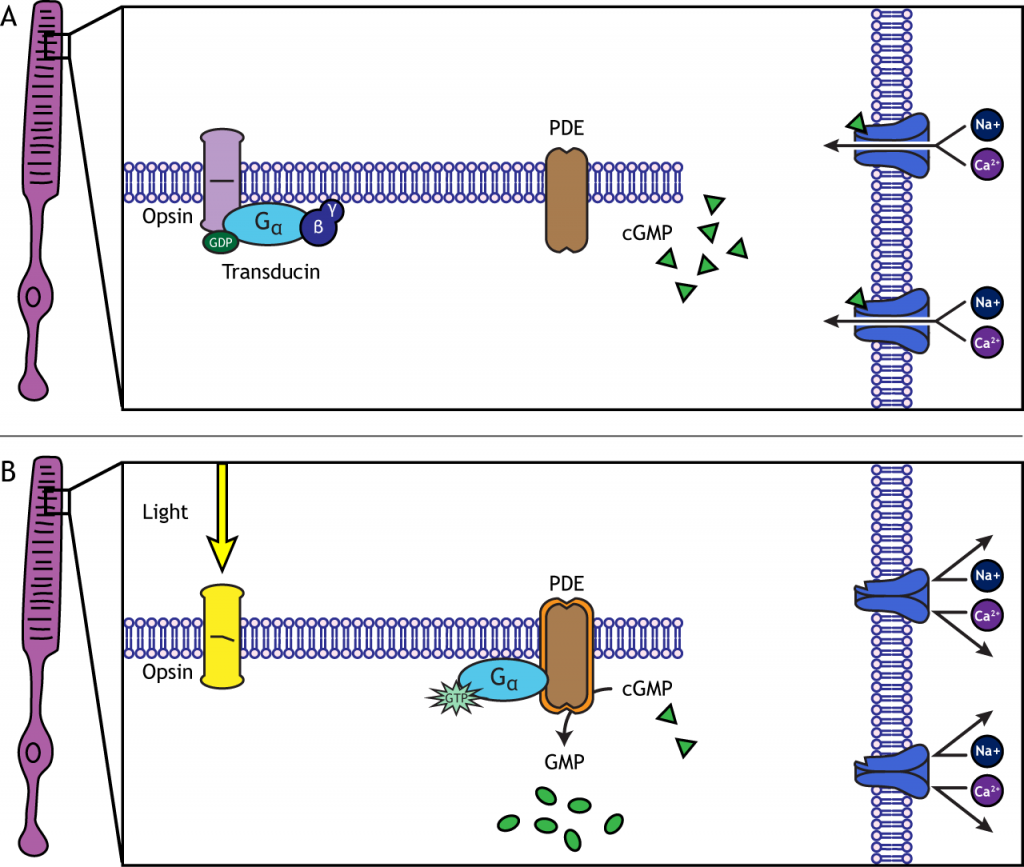