Part 1: Green & Sustainable Chemistry
1 Twelve Principles of Green Chemistry
In the early 1960s, prominent reports and crises[1] prompted concern over the effects of chemical substances on the health of the environment and human populations. The logic behind disposal of chemical substances at this time had been “dilution as the solution to pollution,” which (now debunked) posited that decreasing the substance’s concentration would mitigate the harmful impact. The public outcry and expense of remediation of these environmental disasters led to legislation to mandate the manufacture, use, and disposal of chemical substances. By the 1990s, it was clear that the solution was to prevent pollution at the source, reducing the need for treatment of chemical wastes. One such type of pollution prevention scheme is the approach to synthesis that has been dubbed “Green Chemistry”[2]. The focus of this approach is to design and redesign chemicals and/or processes to prevent pollution and favor chemicals that pose less of a risk to human and environmental health. The ultimate goal of this “benign” approach to chemical synthesis is “to design synthetic methodologies that reduce or eliminate the use or generation of toxic feedstocks [reagent sources], by-products, [and] solvents.”
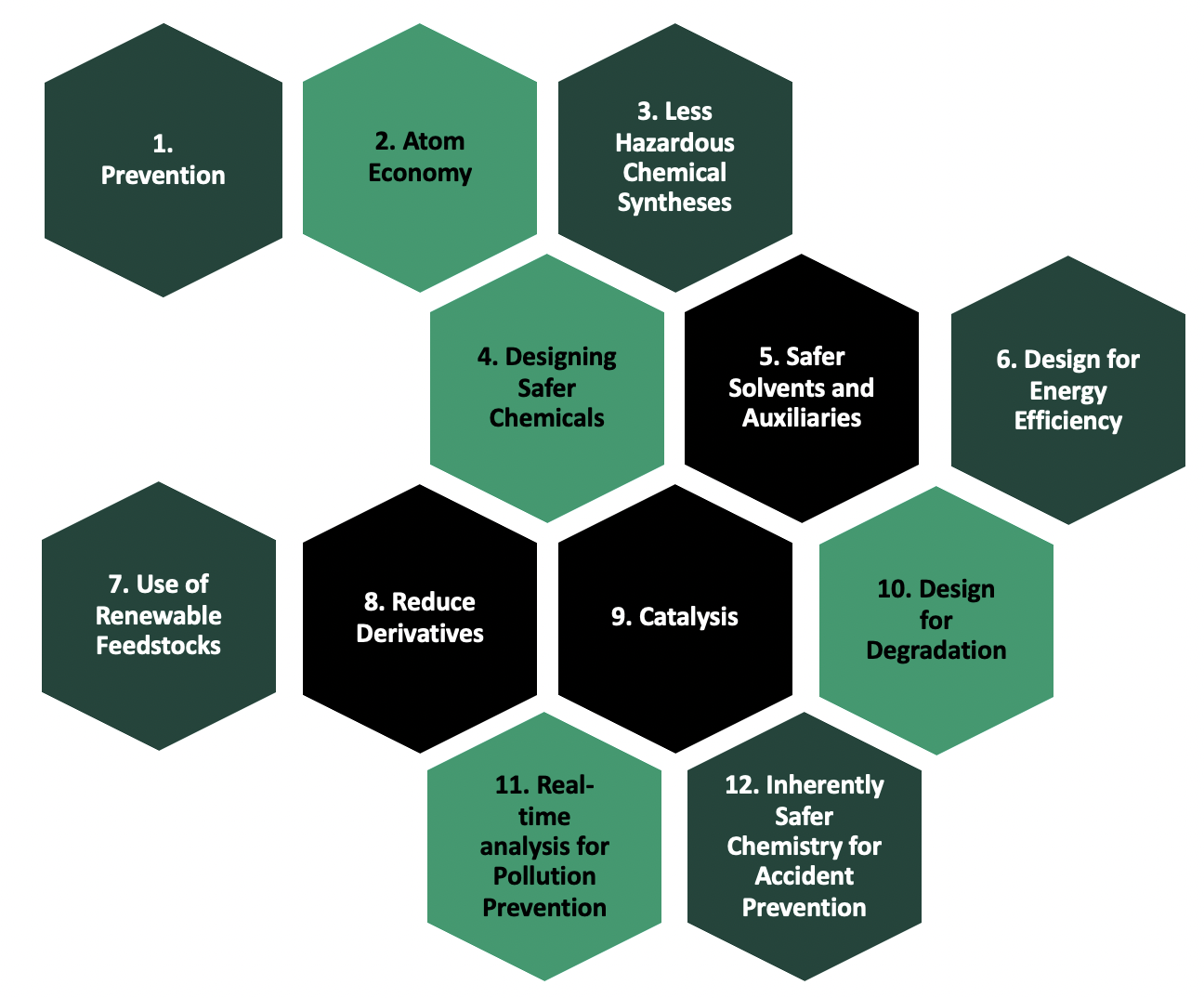
Pictured in Figure 1, the culmination of innovation in the 1990s was the 12 Principles of Green Chemistry. The twelve principles are explained in greater detail below, and the list includes: (1) prevention, (2) atom economy, (3) less hazardous chemical synthesis, (4) designing safer chemicals, (5) using safer solvents and auxiliaries, (6) designing for energy efficiency, (7) using renewable feedstocks, (8) reducing derivatives, (9) catalysis, (10) designing for degradation, (11) real-time analysis of pollution prevention, and (12) inherently safer chemistry for accident prevention.
These 12 principles represent the considerations and strategies that chemists should employ to analyze and optimize a chemical process as well as the chemicals throughout the entire life cycle. We will explore these principles, their associated metrics, and the broader impacts of the chemistry in the case studies associated with this course. The individual green chemistry principles are enumerated below, but they follow overarching themes of safety, efficiency, and eco-consciousness through the prevention of pollution and attention to renewable sources of materials.
Principle 1: Waste Prevention
It is better to prevent waste than to treat or clean up waste after it has been created. [3]
This principle embodies much of the clarity gained in the 1990s around the problem with pollution. Rather than expensive and less effective remediation projects, it is far more efficient to design processes to be benign, especially by preventing the production of waste. While simple and clear, such an endeavor requires careful planning and analysis of the whole chemical process.
As we will cover in greater detail in the Handling Waste section, waste includes everything but the desired product—excess or used reagents and solvents or consumable materials such as plastic pipettes and weigh paper—but a more expansive way of viewing chemical processes is to consider the generation of waste at all time points in a chemical’s life cycle.
A general Life Cycle for a material is pictured in Figure 2. From a chemical’s extraction to its transformation in industrial processes to its shipping, intended use and end-of-life (reuse, recycling, disposal), there is waste generated at every step in the process.
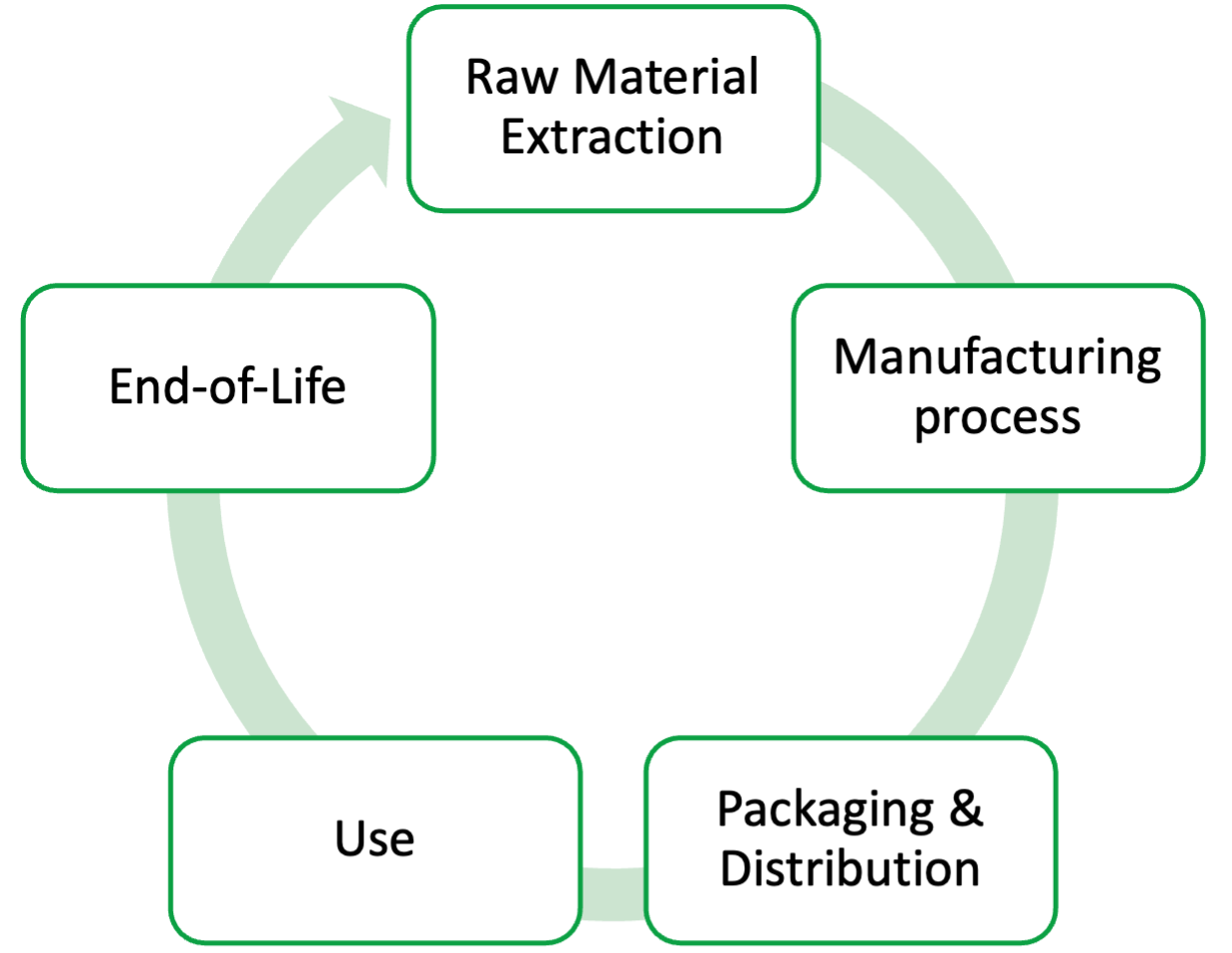
For the sake of the projects in this laboratory course, and for specific chemical processes in the case studies, we will focus on the waste generated by one component of this entire system: the laboratory, specifically at the scale of the laboratory bench. To aid our evaluations of waste generation for various processes, we can employ two metrics: e-factor and process mass intensity (PMI).
E-Factor
One classic metric of waste generation in organic chemistry is the e-factor[4], a ratio of the mass of waste produced in the process to the mass of desired product. This metric is encompassing in that it takes into account the yield of desired product (giving us a sense of the relative cost per unit mass of product) as well as the masses of actual waste: reagents, solvents (including loss of solvent), any auxiliary compounds, and potentially the amount of fuel needed (albeit not an easy thing to calculate). However, because of the perceived “green-ness” of water-based reactions, water used in the process is not included in this calculation.
[latex]e - \textrm{factor} = \frac{\textrm{amount}\:(\textrm{kg})\:\textrm{of}\:\textrm{waste}}{\textrm{amount}\:(\textrm{kg})\:\textrm{of}\:\textrm{desired}\:\textrm{product}}[/latex]
A higher e-factor indicates more waste generation and a greater environmental impact. Ideally, the e-factor would be zero. Oil refining industries report e-factors <0.1 whereas pharmaceutical industries report a range from 25-100.7
Process Mass Intensity (PMI)
This metric provides a more fine-grained analysis of the individual steps within a chemical process. The materials included in this mass ratio include reactants, solvents for reaction and purification, and catalysts.[5] As with e-factor, a lower PMI is desirable; the lowest possible PMI is 1. PMI can be calculated using the equation below.
[latex]PMI = \frac{\textrm{total}\:(\textrm{mass})\:\textrm{in}\:\textrm{a}\:\textrm{process}\:\textrm{or}\:\textrm{process}\:\textrm{step}\:\textrm{(kg)}}{\textrm{mass}\:(\textrm{of})\:\textrm{product}\:\textrm{(kg)}}[/latex]
Overall, both metrics assess the amount of waste generated relative to the desired product yield. Neither metric makes a distinction about the hazards and relative risks of the waste in these calculations, which is an assessment that we will discuss more in the Classifying Hazards section.
Principle 2: Atom Economy
Synthetic methods should be designed to maximize incorporation of all materials used in the process into the final product.[6]
Atom economy is both a principle and a metric by which to judge a chemical process. As a principle, starting materials that closely resemble the structure of desired product will produce the highest atom economy, maximizing the number of atoms of starting materials in the desired product and therefore minimizing by-products as waste.
Atom Economy
The atom economy metric[7] is expressed as a percentage of the weight of all atoms of the reactants used (i.e., incorporated into the desired product) to the sum of the weights of all reagents. In an efficient, atom-economical reaction, this metric would be 100%, indicating that every atom of the reagents was somehow incorporated into the desired product. (Recall that FW is the formula weight, the sum of atomic weights of all atoms in a given chemical formula).
[latex]\textrm{Atom}\:\textrm{Economy}\:\textrm{(%)} = \frac{\textrm{FW}\:\textrm{of}\:\textrm{atoms}\:\textrm{utilized}}{\:\textrm{sum}\:\textrm{of}\:\textrm{FW}\:\textrm{of}\:\textrm{all}\:\textrm{reactants}}[/latex]
In your argumentation for reporting your findings for each lab project, you may be asked to calculate atom economy as a metric to judge reaction schemes. This metric could be used in contrast to one you are more familiar with: percent yield.
[latex]\textrm{Percent}\:\textrm{Yield}\:\textrm{(%)} = \frac{\textrm{actual}\:\textrm{mass}\:\textrm{of}\:\textrm{desired}\:\textrm{product}\:\textrm{(g)}}{\:\textrm{theoretical}\:\textrm{mass}\:\textrm{possible}\:\textrm{based}\:\textrm{on}\:\textrm{reaction}\:\textrm{stoichiometry}\:\textrm{(g)}}[/latex]
However, notice that yield doesn’t incorporate the masses of any material besides the desired product, for example, catalyst. However, use of catalyst will impact the atom economy value of a synthetic route. Relying on this metric alone, one could have a reaction that is very efficient at generating a desired product, but inefficiently incorporate starting material into that product which leaves much of the starting mass to be relegated as waste.
Principle 3: Less Hazardous Chemical Syntheses
Wherever practicable, synthetic methods should be designed to use and generate substances that possess little-to-no toxicity to human health and the environment.[8]
The metrics discussed in Principles 1 and 2 help serve as benchmarks for efficiency and minimization of the quantity of waste; this principle attends to the identity of that waste, its inherent hazards, and the toxicity it may pose to human health and the environment. Although, as noted by the “wherever practicable”, we recognize that there may not be preferred substitutions for certain reagents, solvents, or auxiliaries. In cases where a substitution is possible, there are practical concerns such as efficiency and cost to consider.
For the context of this course, several of these decisions have already been incorporated into the design of the projects your team will engage in. There will be opportunities for your team to engage in green decision-making surrounding solvent use for certain techniques. This Principle 5: Safer Solvents and Auxiliaries will provide you with a Greener Solvent List to guide those decisions.
EcoScale
The primary source material of hazard information for chemicals used and generated will be discussed further in Classifying Hazards in the next section. The metric we will use to incorporate hazard information into our assessments will be EcoScale.[9] EcoScale take penalty points off six categories: yield, price of reaction components, safety, technical setup, temperature/time and workup and purification, as shown in Table 1. A high EcoScale score is preferred. EcoScale metric favors high yields, low-cost, less reaction time and safer reaction conditions with low energy consumption and easier processing methods. The penalty points to calculate the EcoScale is summarized in the table below. Several online EcoScale calculators are available including: The Ecoscale
Parameter |
Penalty points |
---|---|
1. Yield |
(100 – %yield)/2 |
2. Price of reaction components (to obtain 10 mmol of end product) |
|
Inexpensive (< $10) |
0 |
Expensive (> $10 and < $50) |
3 |
Very expensive (> $50) |
5 |
3. Safetya |
|
N (dangerous for environment) |
5 |
T (toxic) |
5 |
F (highly flammable) |
5 |
E (explosive) |
10 |
F+ (extremely flammable) |
10 |
T+ (extremely toxic) |
10 |
4. Technical setup |
|
Common setup |
0 |
Instruments for controlled addition of chemicalsb |
1 |
Unconventional activation techniquec |
2 |
Pressure equipment, > 1 atmd |
3 |
Any additional special glassware |
1 |
(Inert) gas atmosphere |
1 |
Glove box |
3 |
5. Temperature/time |
|
Room temperature, < 1 h |
0 |
Room temperature, < 24 h |
1 |
Heating, < 1 h |
2 |
Heating, > 1 h |
3 |
Cooling to 0°C |
4 |
Cooling, < 0°C |
5 |
6. Workup and purification |
|
None |
0 |
Cooling to room temperature |
0 |
Adding solvent |
0 |
Simple filtration |
0 |
Removal of solvent with bp < 150°C |
0 |
Crystallization and filtration |
1 |
Removal of solvent with bp > 150°C |
2 |
Solid phase extraction |
2 |
Distillation |
3 |
Sublimation |
3 |
Liquid-liquid extractione |
3 |
Classical chromatography |
10 |
Principle 4: Designing Safer Chemicals
Chemical products should be designed to preserve efficacy of function while reducing toxicity.[11]
From a green chemistry perspective, safer chemicals are those that are designed and produced with a focus on minimizing negative impacts on the environment and on human health. Safer chemicals exhibit characteristics such as low toxicity, safe handling and use, high degradability, minimal waster generation and low energy requirements during production, etc.
Designing chemicals is beyond the scope of this course, but one goal of this course is to provide opportunities to use these principles and metrics to evaluate sustainability problems. As we will unpack further in the section Toxicology and Green & Sustainable Chemistry, careful consideration of the hazards inherent to certain chemicals (and processes) and how these chemicals interact with human health and the environment can be a powerful tool for identifying necessary areas for change and innovation. Tasks within this learning goal will incorporate some basic ideas of toxicology and environmental science.
Principle 5: Safer Solvents and Auxiliaries
The use of auxiliary substances (e.g., solvents, separation agents, etc.) should be made unnecessary wherever possible and innocuous when used.[12]
As you’ve covered in lecture, the solvent has an important role in the rate of the reaction as it interacts with the reactants. After the reaction, the solvent and other auxiliary compounds play an important role in the workup. The workup includes all process steps after the reaction step with the broad goals of separating, purifying, and confirming purity and yield of the target product. As you may discover in your projects and case study #1, the workup can often be the step in the entire chemical process that is the least green, requiring energy input and/or toxic or hazardous solvents to separate the desired product from by-products or impurities. If practicable, eliminating solvents altogether and making use of mechano-chemistry or other solvent-free setups is highly desirable.
On basic level, as this course was designed to feature Green and Sustainable Chemistry, a lot of substitutions for safer, less hazardous chemicals have already been made for you. However, there are explicit opportunities for you to make sustainable decisions within the context of your investigations. When appropriate, you will be directed to choose a solvent with the characteristics (polarity, basicity, etc.) from the Greener Solvent List in Table 2.
Greener Solvent List
Table 2. Greener Solvent List from Beyond Benign (V.1 March 2020)

As noted, there’s no universal approach to solvent selection. In the sections following, instructions for how to test solvents on a small scale are given. To help interpret this solvent guide table, each bullet point below discusses a line in Table 2.
- If seeking to replace hexane(s) and pentane as nonpolar solvents, this solvent guide suggests heptane and isooctane as problematic, yet greener alternatives.
- If looking to replace Dimethylformamide (DMF), dimethylacetamide (DMAc), or N-methyl-2-pyrroliodone (NMP), the greenest alternatives available are cyrene, cyclopentyl methyl ether (CPME), or dimethyl carbonate (DMC); more problematic alternatives include dimethyl sulfoxide (DMSO) and acetronitrile (MeCN).
- If looking to replace Tetrahydrofuran (THF), the best alternative is CPME, although Methyl tert-butyl ether (MTBE) or 2-methyltetrahydrofuran (2-MeTHF) are viable yet problematic alternatives.
- If trying to replace Diethyl ether (Et2O) or Di-isopropyl ether, the best alternative is CPME, although Methyl tert-butyl ether (MTBE) or 2-methyltetrahydrofuran (2-MeTHF) are viable yet problematic alternatives.
- If trying to replace Dimethoxyethane (DME) or dioxane, the best alternative is CPME, although Methyl tert-butyl ether (MTBE) or 2-methyltetrahydrofuran (2-MeTHF) are viable yet problematic alternatives.
- For highly hazardous solvents chloroform (CHCl3), dichloroethane (DCE), or carbon tetrachloride (CCl4), the only alternative is also undesirable, dichloromethane (DCM).
- For replacing pyridine in applications as a base, triethylamine (Et3N) is a problematic alternative.
- DCM is commonly used in extractions despite its undesirability, the greenest alternative is ethyl acetate (EtOAc), but MTBE, 2-MeTHF, or toluene are viable yet problematic alternatives.
- For DCM’s use in chromatography, the ideal replacement would be a 3:1 ratio of EtOAc to ethanol (EtOH); a 50:50 mixture of EtOAc to heptane could be used, although is less green.
- Highly hazardous benzene is best replaced by toluene, which is a problematic alternative.
- Finally, one can “green” their procedure by replacing acetone for ethyl lactate or DMC, or with EtOH in the context of washing the product.
Principle 6: Design for Energy Efficiency
Energy requirements should be recognized for their environmental and economic impacts and should be minimized. Synthetic methods should be conducted at ambient temperature and pressure.[13]
As noted in the previous Principle, there are energy-expensive steps in the workup (and in the reaction step itself) procedures following a reaction. The use of electricity, energy in the form of heat, or water to cool condensing setups all have an economic cost, and this use of energy does contribute to the generation of greenhouse gases (GHGs) and the effects of climate change. While calculating energy usage (and the relative GHG creation) can be tricky in the laboratory classroom, we can prioritize reactions that run at ambient (room) temperatures and pressures. Energy consumption in an academic environment may be negligible. The energy usage and associated cost can be significant on a large industrial scale, while extreme temperature and pressure conditions are prevalent in various industries. Design for energy efficiency is imperative to reduce economic and environmental costs.
Principle 7: Use of Renewable Feedstocks
A raw material or feedstock should be renewable rather than depleting whenever technically and economically practicable.[14]
As noted in the Life Cycle in Figure 2, an important sustainable consideration is the source of the chemicals and materials we use at the bench. Historically, since the 1800s, chemicals have been refined and transformed from petroleum. Most chemicals we use are extracted from oil, a non-renewable resource! Even hydrogen peroxide (H2O2)—which is considered a greener oxidizing agent, especially compared to heavy metals such as chromium or manganese—derives from oil refineries rather than one of the pharmacy shelves at the store.
This principle highlights the urgent need to develop renewable sources of chemicals. However, as we will explore in Case Study, some renewable sources are also food sources (such as sugar cane) and removing those materials from the food supply to serve the chemical industry could have detrimental impacts on UN Sustainable Development Goal #2 – Zero Hunger.[15]
The 17 UN Sustainable Development Goals (SDGs), shown in Figure 3, was adopted by the United Nations Member State in 2015, calling for action by all countries in a global partnership towards peace and prosperity for people and the planet, now and into the future.
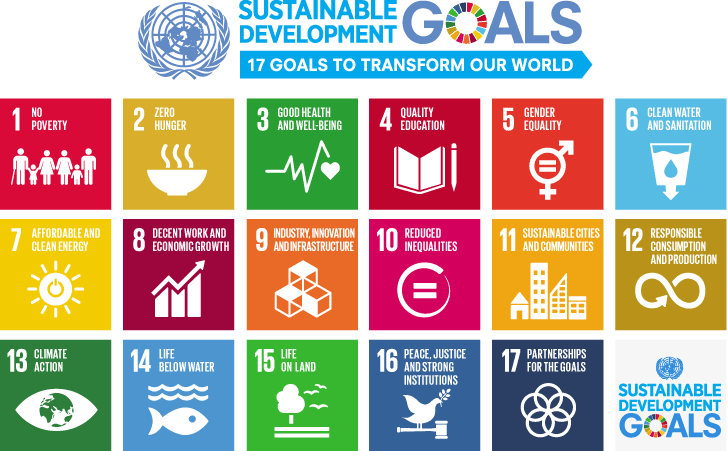
Principle 8: Reduce Derivatives
Unnecessary derivatization (use of blocking groups, protection/deprotection, temporary modification of physical/chemical processes) should be minimized or avoided if possible, because such steps require additional reagents and can generate waste.[16]
In research and industrial laboratories, the use of blocking, protecting/deprotecting, or other temporary modifications to reagent molecules is common to drive the reaction in a desired direction. This derivatization of molecules in a reaction scheme is useful, but it introduces more steps and uses more energy and material resources.
Designing reactions and processes to avoid derivatization is beyond the scope of this course. However, as you take future chemistry, biochemistry, or biology courses, be aware that the solution to this issue likely lies in enzymatic or biological processes to carry out these chemical transformations.[17]
Principle 9: Catalysis
Catalytic reagents (as selective as possible) are superior to stoichiometric reagents.[18]
In the general and organic chemistry lectures, we have explored the effect of a catalyst on a reaction’s rate. These substances—chemicals, enzymes, or even surfaces—accelerate a reaction without serving as a reactant by providing an alternate pathway for the reaction to occur, resulting in a lower activation energy than the uncatalyzed pathway (which is the “stoichiometric” ratio of reagents).[19] With this lower activation energy, ambient temperatures (and pressures) are more feasible, and a catalyzed-reaction pathway may be more selective, require fewer process steps, require less reagent, produce less waste, and result in a higher atom economy.[20]
Principle 10: Design for Degradation
Chemical products should be designed so that at the end of their function they break down into innocuous degradation products and do not persist in the environment.[21]
This green principle challenges molecular designers (expert chemists) to consider end-of-life when choosing a target molecule. Referring to the Life Cycle in Figure 2, we can leverage the structure-property relationships to design molecular targets that will be effective for their use but at the end-of-life stage will “readily degrade and [won’t] persist and accumulate in the environment.”[22]
While the work of designing products to be effective yet degradable is beyond the scope of this course, we will use this principle in the Case Studies as we explore current, urgent sustainability problems that illustrate how useful chemical and material products can have long-lasting and negative effects in their end-of-life.
Principle 11: Real-Time Analysis for Pollution Prevention
Analytical methodologies need to be further developed to allow for real-time, in-process monitoring and control prior to the formation of hazardous substances.[23]
While this principle suggests an area of innovation and investment for industry, pollution prevention is a challenge if we don’t have accurate and timely data to evaluate processes. As you may find as your team collects data for the green metrics and tools described above, some data may be challenging to collect with accuracy and precision.
Principle 12: Inherently Safer Chemistry for Accident Prevention
Substances and the form of a substance used in a chemical process should be chosen to minimize the potential for chemical accidents, including releases, explosions, and fires.[24]
This principle is well-aligned with Principle 3: Less Hazardous Chemical Syntheses and the RAMP safety approach described in the next section. Designing and carrying out greener chemical investigations requires attention to minimizing risk of hazards by choosing substances that are more benign. Coupled with a culture of safety and thoughtful preparation for emergencies, the green chemistry laboratory is inherently safer.
Other Metrics
Green Chemistry is a rapidly evolving field with new developments emerging every day. Other green chemistry metrics are available to evaluate the greenness of a production process, each offering unique insights into different aspects of environmental impact and sustainability.
- Lice Cycle Assessment (LCA): Focuses on evaluating the environmental impacts associated with all stages of a product’s life, from raw material extraction through manufacturing, distribution, use, and disposal or recycling, in the hopes of improving environmental performance.
-
Reaction Mass Efficiency (RME): Provides a quantitative measure of how effectively starting materials are converted into the desired product, considering both the yield and the mass of reactants used. The calculation is typically expresses in percentage.
- DOZN quantitative Green Chemistry Evaluator: A quantitative, industry-first tool that uses the 12 Principles of Green Chemistry for comparing the relative greenness of similar chemicals, synthetic routes and chemical processes. More information is available via: DOZN™ Quantitative Green Chemistry Evaluator.
- And more…
By employing a combination of the metrics, chemists and industries can make informed decisions to design and implement greener processes that align with sustainability goals and regulatory requirements.
Three Themes on Green and Sustainable Chemistry
These twelve principles could be grouped into three overarching themes: a commitment to efficiency, safety, and overall benign chemistry. As you plan and carry out your investigations for each project, the planning questions will guide you to consider the efficiency—in terms of e-factor, PMI, and atom economy—benign-ness of the substances in the reaction—using the hazard information and EcoScale—and uphold a culture of safety within your team and course. The next section will detail how a RAMP approach to safety will be enacted in the planning process.
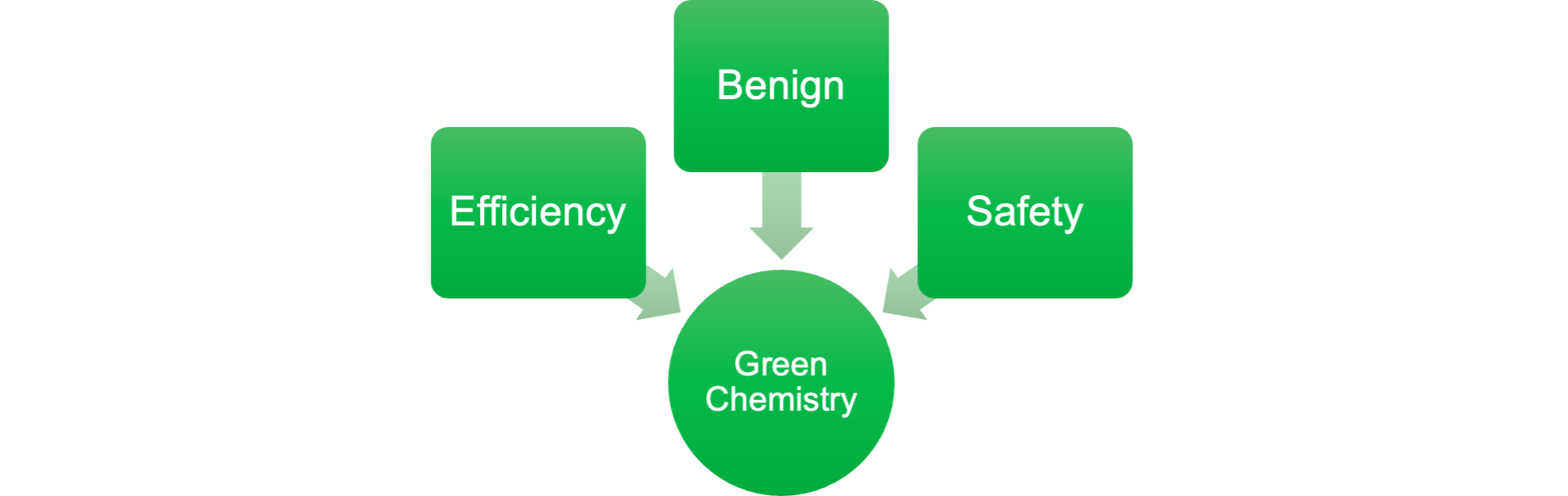
- Warner, John & Cannon, Amy & Dye, Kevin. (2004). Green Chemistry. Environmental Impact Assessment Review. 24. 775-799. 10.1016/j.eiar.2004.06.006. ↵
- Developed by Paul Anastas and John Warner. Anastas, P. T.; Warner, J. C. Green Chemistry: Theory and Practice. Oxford University Press: New York, 1998. More information available online through the American Chemical Society’s Green Chemistry Institute. ↵
- This builds on work by Berkeley W. Cue, Jr., Ph.D., BWC Pharma Consulting, LLC. 12 Principles of Green Chemistry ↵
- Sheldon, R. A. “The E Factor: fifteen years on” Green Chem. 2007, 9, 1273-1283, doi: 10.1039/B713736M ↵
- Jiménez-González, C. et al “Using the Right Green Yardstick: Why Process Mass Intensity Is Used in the Pharmaceutical Industry to Drive More Sustainable Process” Org. Process Res. Dev. 2011, 15 (4), 912-917, doi: 10.1021/op200097d ↵
- This builds on work by Michael Cann, Ph.D., Professor of Chemistry, University of Scranton. 12 Principles of Green Chemistry ↵
- Trost, B. “The Atom Economy-A Search for Synthetic Efficiency” Science 1991, (254), pp. 1471-1477. ↵
- This builds on work by by David J. C. Constable, Ph.D., Director, ACS Green Chemistry Institute® 12 Principles of Green Chemistry ↵
- Aken, K. V.; Strekowski, L.; Patiny, L. Beilstein J Org Chem 2006, 2 (3), doi: 10.1186/1860-5397-2-3 ↵
- Van Aken, K.; Strekowski, L.; Patiny, L. Beilstein J. Org. Chem. 2006, 2, No. 3. doi:10.1186/1860-5397-2-3, CC BY 2.0 DEED ↵
- This builds on work by Nicholas D. Anastas, Ph.D., U.S. Environmental Protection Agency- New England 12 Principles of Green Chemistry ↵
- This builds on work by Dr. Concepcíon (Conchita) Jiménez-González, Director, Operational Sustainability, GlaxoSmithKline 12 Principles of Green Chemistry ↵
- This builds on work by Dr. David Constable, Director, ACS Green Chemistry Institute® 12 Principles of Green Chemistry ↵
- This builds on work by Dr. Richard Wool, Professor of Chemical and Biomolecular Engineering and Director of the Affordable Composites from Renewable Materials program, University of Delaware. 12 Principles of Green Chemistry ↵
- United Nations Sustainable Development Goals (SDGs), 2015, United Nations Sustainable Development ↵
- This builds on work by Peter J. Dunn, Green Chemistry Lead, Pfizer 12 Principles of Green Chemistry ↵
- Beyond Benign Green Chemistry: Principles and Lab Practices 2020 A Guide to Green Chemistry Experiments for Undergraduate Organic Chemistry Labs (PDF) ↵
- This builds on work by Roger A. Sheldon, Ph.D., Emeritus Professor of Biocatalysis and Organic Chemistry, Delft University of Technology and CEO of CLEA Technologies B.V. 12 Principles of Green Chemistry ↵
- Cooper, M. M.; Klymkowsky, M. “Chapter 8: How far? How fast?” CLUE: Chemistry, Life, the Universe, and Everything 2019. https://openbooks.lib.msu.edu/clue/ ↵
- Beyond Benign Green Chemistry: Principles and Lab Practices 2020 A Guide to Green Chemistry Experiments for Undergraduate Organic Chemistry Labs (PDF) ↵
- This builds on work by Rich Williams, Founder and President at Environmental Science & Green Chemistry Consulting, LLC 12 Principles of Green Chemistry ↵
- Beyond Benign Green Chemistry: Principles and Lab Practices 2020, p. 13, A Guide to Green Chemistry Experiments for Undergraduate Organic Chemistry Labs (PDF) ↵
- This builds on work by Douglas Raynie, Assistant Professor, Chemistry & Biochemistry, South Dakota State University. 12 Principles of Green Chemistry ↵
- This builds on work by Shelly Bradley, Campus Chemical Compliance Director, Hendrix College; Dr. David C. Finster, Professor of Chemistry, Wittenberg University; and Dr. Tom Goodwin, Elbert L. Fausett Professor of Chemistry, Hendrix College. 12 Principles of Green Chemistry ↵